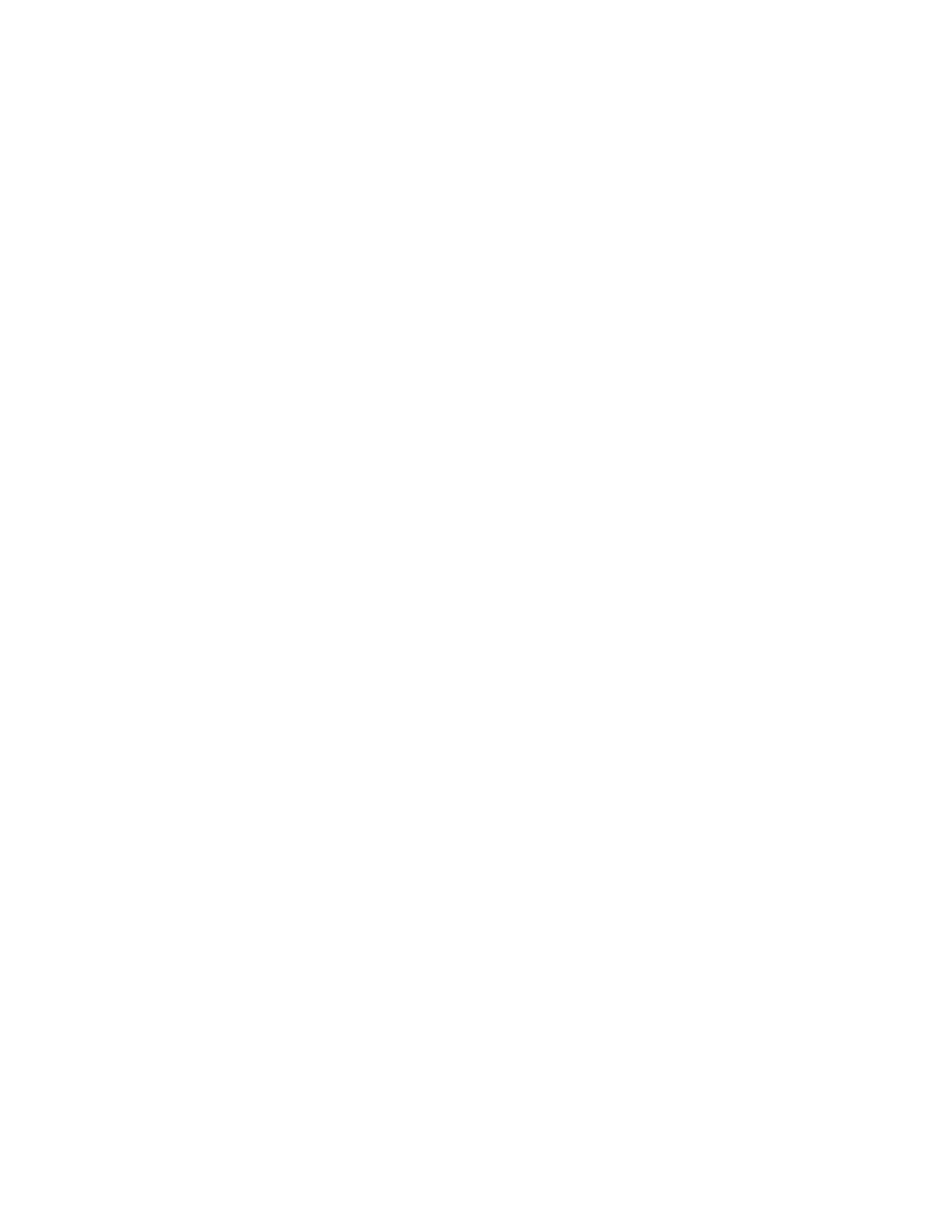
Table des matières
Résumé ................................................................................................................ iii
Table des matières ................................................................................................ v
Liste des abréviations ........................................................................................... ix
Liste des tableaux ............................................................................................... xiii
Liste des figures ................................................................................................... xv
Remerciements .................................................................................................. xix
Avant-Propos ................................................................................................... xxiii
Chapitre 1 ..................................................... 1
Chapitre 1. Introduction ....................................................................................... 3
1.1. La réponse aux cassures double-brin de l'ADN ................................................................... 4
1.1.1. La surveillance du génome: les points de contrôle et la détection des cassures ............. 4
1.1.1.1. Les acteurs moléculaires des points de contrôle ........................................................ 6
1.1.1.2. Le point de contrôle G1/S .......................................................................................... 7
1.1.1.3. Le point de contrôle intra-S ........................................................................................ 8
1.1.1.4. Le point de contrôle G2/M ......................................................................................... 8
1.1.1.5. Le point de contrôle de restauration de la réplication ............................................. 10
1.1.1.6. La détection et la signalisation d'une cassure double-brin ...................................... 10
1.1.2. La réparation des cassures double-brin .......................................................................... 13
1.1.2.1. La jonction d'extrémités non-homologues : les voies classique et alternative ........ 14
1.1.2.2. La recombinaison homologue .................................................................................. 17
1.1.2.3. L'appariement des extrémités protubérantes simple-brin ....................................... 20
1.1.3. Les maladies associées à un défaut de la réparation de l'ADN ....................................... 21
1.2. Focus sur certains acteurs de la réparation de l'ADN ....................................................... 23
1.2.1. La recombinase RAD51 ................................................................................................... 23
1.2.2. BRCA1 et BRCA2 .............................................................................................................. 24
1.2.2.1. BRCA1 ...................................................................................................................... 24
1.2.2.2. BRCA2 ...................................................................................................................... 24
1.2.3. PALB2 et les ADN polymérases ....................................................................................... 26
1.2.3.1. Le suppresseur de tumeur PALB2 ............................................................................. 26
1.2.3.2. Les ADN polymérases dans la réparation des dommages à l'ADN .......................... 26
1.3. Les stratégies thérapeutiques contre le cancer utilisant les cassures double-brin ............ 28
1.3.1. Les stratégies thérapeutiques ......................................................................................... 28
1.3.1.1. Les stratégies existantes et leurs perspectives de développement .......................... 28
1.3.1.2. Le principe de létalité synthétique ........................................................................... 29
1.3.2. Les agents thérapeutiques conventionnels .................................................................... 31
1.3.2.1. La radiothérapie ...................................................................................................... 31
1.3.2.2. La chimiothérapie .................................................................................................... 31
1.3.2.3. Les inhibiteurs de topoisomérase ............................................................................ 31
1.3.3. Les nouvelles classes d'agents thérapeutiques en développement ............................... 34
1.3.3.1. Les inhibiteurs de PARP ............................................................................................ 34
1.3.3.2. Les inhibiteurs des senseurs des cassures double-brin et du cycle cellulaire ........... 35
1.3.3.3. Les inhibiteurs du NHEJ ............................................................................................ 36
1.3.3.4. Les inhibiteurs de la Recombinaison Homologue .................................................... 37
1.3.4. Les limites et perspectives des traitements .................................................................... 39
Objectifs ............................................................................................................. 41