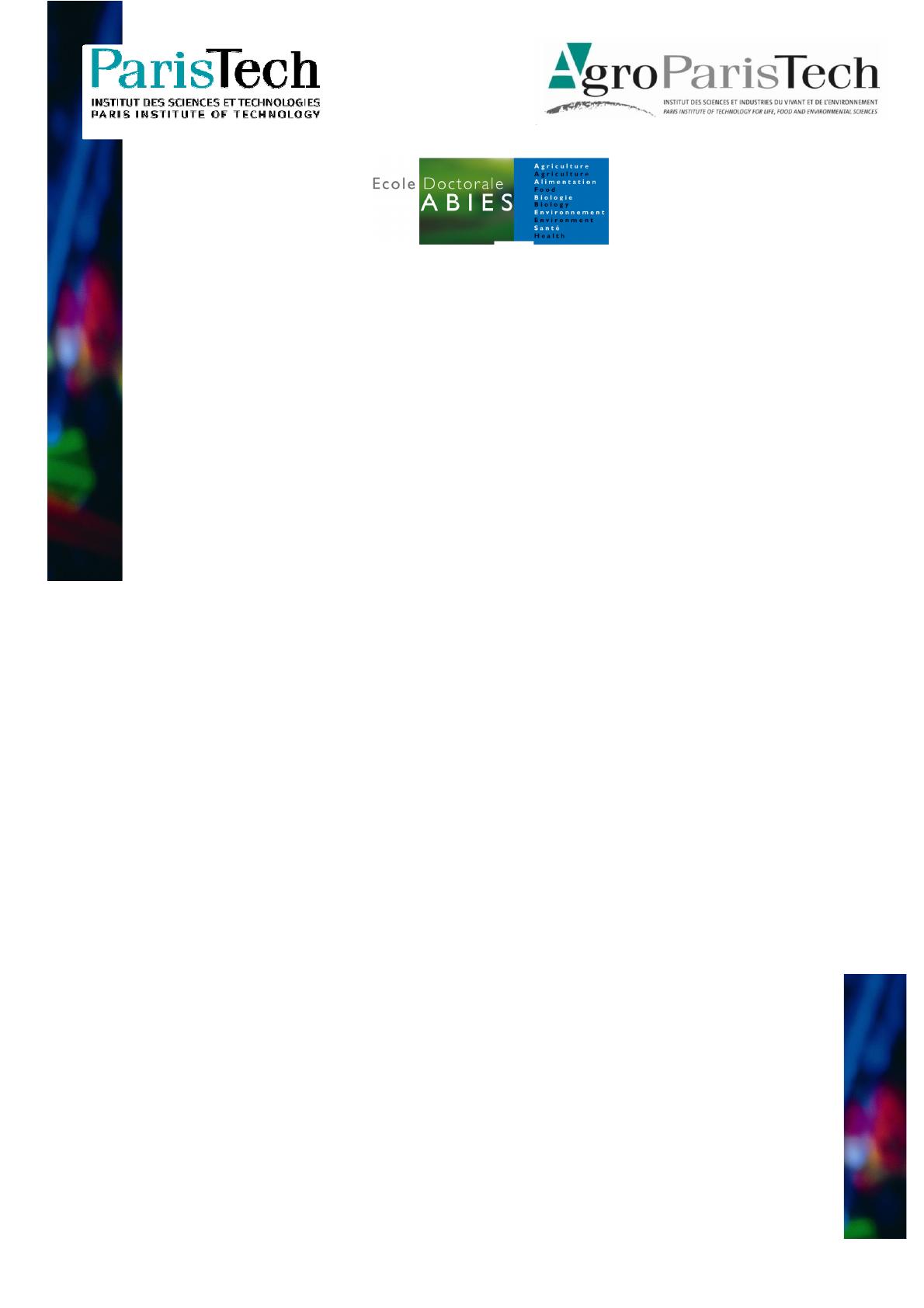
N°: 2009 ENAM XXXX
AgroParisTech
Laboratoire d'Etude des Ressources Forêt-Bois
14 rue Girardet - CS 14216 - 54042 Nancy Cedex - France
présentée et soutenue publiquement par
Rosalinde VAN COUWENBERGHE
18 mars 2011
Effets des facteurs environnementaux sur la distribution
et l’abondance des espèces végétales forestières
aux échelles locales et régionales
T H È S E
pour obtenir le grade de docteur délivré par
L’Institut des Sciences et Industries
du Vivant et de l’Environnement
(AgroParisTech)
Spécialité : Sciences Forestières et du Bois
Directeur de thèse : Jean-Claude GEGOUT
Co-encadrement de la thèse : Catherine COLLET, Eric LACOMBE, Jean-Claude PIERRAT
M. Guillaume DECOCQ,
Pr., Plant biodiversity lab, Université de Picardie Jules Verne
Rapporteur
M. Thomas WOHLGEMUTH,
Dr., Forest Dynamics, WSL Birmensdorf
(CH) Rapporteur
M. Jean-Luc DUPOUEY,
Dr., UMR EEF, INRA Nancy
Président
M. Kris VERHEYEN,
Pr., Vakgroep Bos- en Waterbeheer, Universiteit Gent
(BE) Examinateur
M. Jean-François DHOTE,
Dr., Département Recherche & Développement, ONF
Examinateur
Mme Catherine COLLET,
Dr., UMR LERFoB, INRA Nancy
Co-encadrante
M. Jean-Claude GEGOUT,
Pr., UMR LERFoB, AgroParisTech
Directeur de thèse