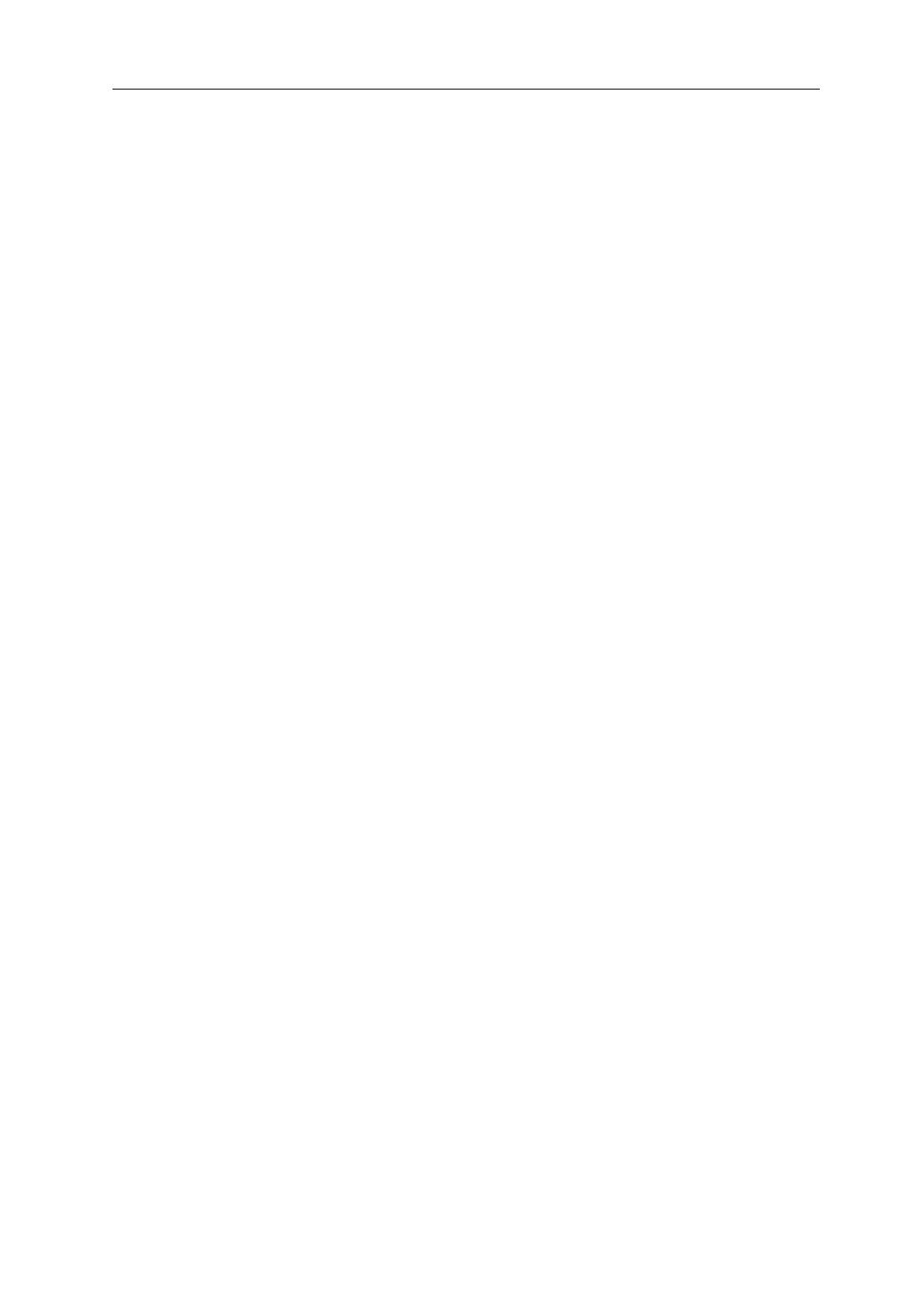
TrimSize 170mm x 244mm Merrifield c04.tex V3 - 07/23/2014 10:31 A.M. Page 77
The Gut Microbiota of Fish 77
study such environmental samples, several culture-independent molecular techniques have
been developed. These methods have allowed the identication of microorganisms without iso-
lation and the determination of the phylogenetic afliation of community members, revealing
the enormous extent of microbial diversity. The analysis of DNA extracted directly from a com-
plex environmental sample provides a powerful and relatively bias-free alternative approach
towards characterizing a microbial community (Nayak 2010). Typically, fragments of 16S or
18S ribosomal genes are selectively amplied by PCR to provide information on prokaryotic
and eukaryotic communities, respectively (Navarrete et al. 2010a). Patterns of diversity and
relative abundance of amplied DNA fragments can then be assayed using several strategies
(see Chapter 5). Massive sequencing technologies have revolutionized this eld by allowing
direct sequencing of millions of DNA molecules from a single sample (Qin et al. 2010). It is
thus now possible to obtain unbiased qualitative and quantitative reconstructions of complete
microbial communities – including both cultivable and uncultivable representatives – within
reasonable time frames and at affordable cost. Molecular techniques have been successfully
applied in dozens of studies proling the GI microbial communities of sh. Several attempts
have been made to describe the microbiota in a number of important aquacultured sh species.
Molecular methods based on PCR amplication of DNA extracted from frozen samples have
typically been the favoured approach and have proven to be efcient in studying the GI bac-
terial community of shes (Grifths et al. 2001; Jensen et al. 2004; Romero and Navarrete
2006; Hovda et al. 2007; Kim et al. 2007). Recently, studies have begun to analyse the sh
gut microbiota using massive sequencing strategies (e.g. van Kessel et al. 2011; Roeselers
et al. 2011; Desai et al. 2012; Wu et al. 2012). It is anticipated that as this technology becomes
more accessible it will signicantly improve our knowledge of the sh gut microbiota, enabling
identication of the rare biosphere and community metabolic pathways.
The ultimate goal of these studies is to provide a scientic basis for developing effective
strategies for manipulating gut microbial communities to promote animal health and improve
productivity. To achieve this goal, the principles governing microbiota composition (assembly)
and maintenance within the intestine must be understood. The vast majority of these studies
have focused on the bacterial communities and to a lesser extent yeast; very little information
is available for the viral, archaean and protozoan populations in the GI tract of sh.
4.1.1 Current knowledge of the gut microbiota in fish
Our current knowledge of the microbiota composition is derived from a compilation of
information in numerous reports; most of them focused on farmed sh, and among these the
salmonids have received much attention. Figure 4.2 summarizes the most commonly reported
bacterial phyla in salmonids, based on the review of Nayak (2010). Proteobacteria and
Firmicutes are the most frequently reported phyla in the salmonid gut microbiota, suggesting
that members of these bacterial classes are especially well adapted to conditions in the sh
intestine or their surrounding aquatic environment. Interestingly, studies in salmonids show
that some particular bacterial genera can be predominant in the microbiota composition; for
example, Pseudomonas can represent more than 60% of the community when ribosomal
amplicons are cloned and sequenced (Navarrete et al. 2009). The dominance of a particular
bacterial group has been observed in salmonid guts using similar culture-independent meth-
ods. Holben et al. (2002) reported that some genera were highly abundant in reared Atlantic
salmon from two different locations: in a Scottish hatchery, Mycoplasma corresponded to
81% of clones retrieved, whereas in a Norwegian hatchery, Acinetobacter accounted for 55%.