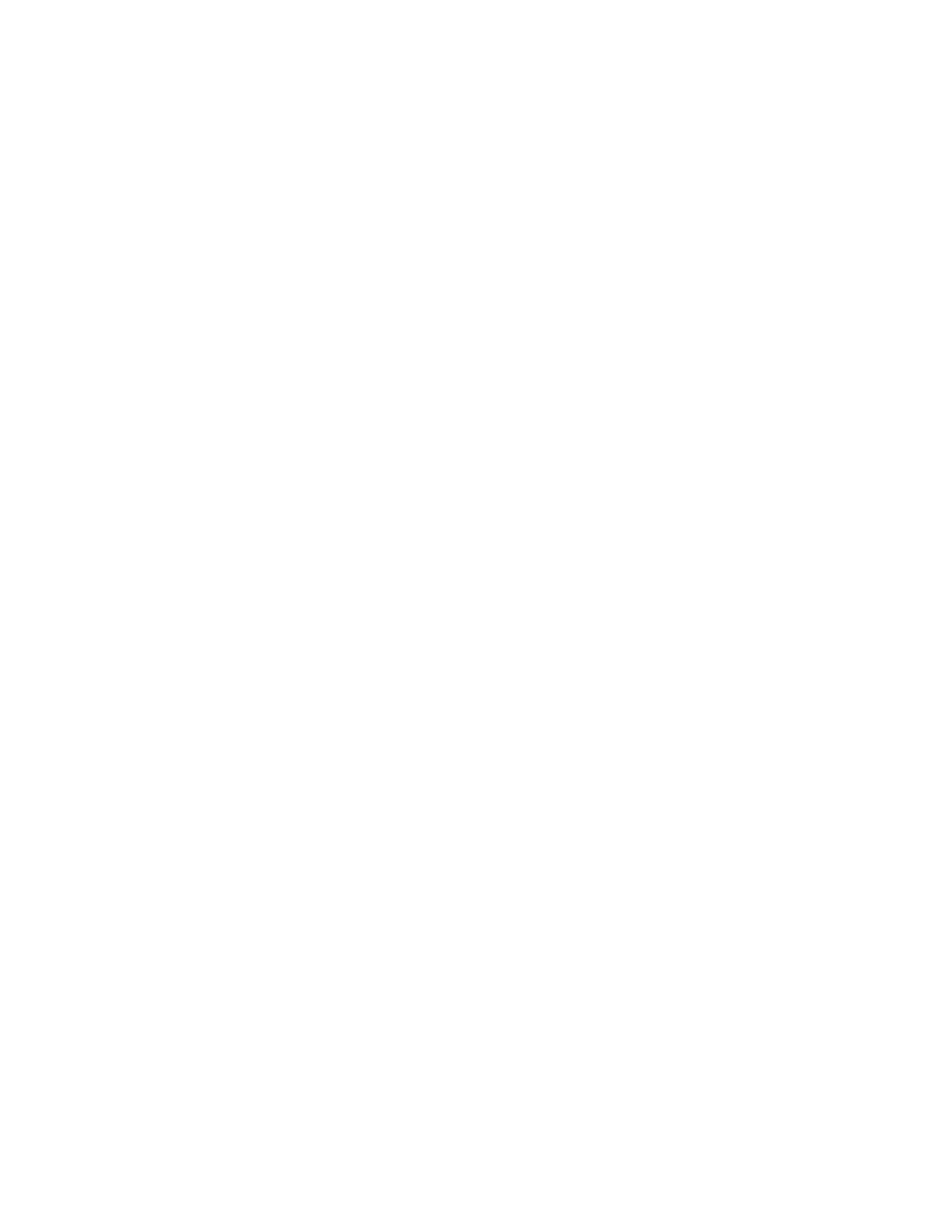
Résumé
Malgré l’amélioration des techniques de procréation médicalement assistée (PMA), les
données recensées depuis 40 ans montrent un faible taux de gestation après transfert
embryonnaire et une incidence élevée de certains syndromes périnataux. Parmi les causes de
l’insuccès de la PMA, les conditions de culture de l’embryon sont sous-optimales pour le
développement normal précoce, occasionnant différents stress qui affectent la qualité de
l’embryon et sa compétence à produire une gestation. Afin de mieux comprendre l’impact de
la PMA sur la qualité embryonnaire, des analyses de micro-puce ont montré des
changements dans l’expression de plusieurs centaines de gènes chez les embryons produits
par culture in vitro en comparaison à ceux produits in vivo. Cependant, les changements
transcriptomiques spécifiquement associés à la baisse de qualité embryonnaire restent encore
indéterminés. En hypothèse, nous supposons que l’étude des différences transcriptomiques
résultant spécifiquement du stress de la culture permette de déterminer les profiles
d’expression génique directement associés à la mauvaise qualité des embryons en culture.
Dans ce contexte, nos objectifs consistaient à moduler le niveau de stress en culture afin
d’affecter la survie embryonnaire, puis de comparer les gènes différentiellement exprimés
entre embryons contrôles et embryons stressés (analyse par miro-puce et RT-qPCR). Pour ce
faire, l’exposition à un stress énergétique, oxydatif ou lipidique a été utilisée séparément
pour départager les différents effets de la culture sur le développement de l’embryon bovin.
Les résultats de ce projet ont mis en évidence l’impact progressif du stress énergétique en
culture sur le métabolisme de l’effet Warburg, un processus développemental permettant une
adaptation pathologique aux dysfonctions mitochondriales. Par la suite, l’impact du stress
oxydatif a révélé des réactions inflammatoires et fibrotiques en association à la baisse de
qualité embryonnaire. Enfin, l’impact du sérum et des lipides s’est traduit par un profil
indiquant des perturbations inflammatoires et métaboliques, complétant notre étude des
mécanismes impliqués dans la réponse au stress de la culture. En conclusion, ce projet a
permis de caractériser des bio-marqueurs récurrents du stress embryonnaire chez le bovin,
ouvrant à des perspectives du diagnostique de la viabilité embryonnaire et du développement
d’alternatives pour mieux cultiver les embryons précoces.