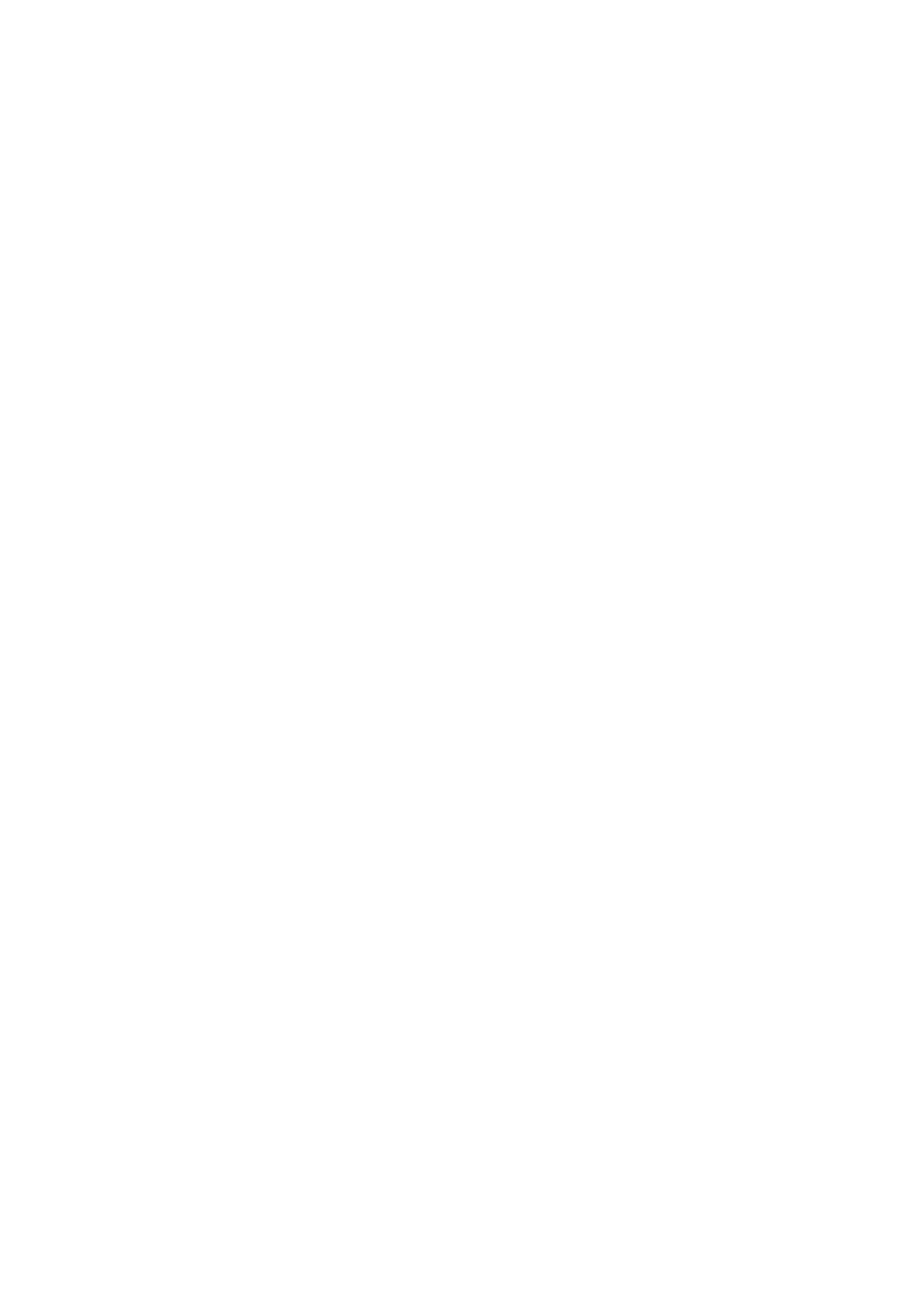
Remerciements
Après ces trois ans et demi passés au Laboratoire d’Astrophysique de Toulouse-Tarbes
puis à l’Institut de Recherche en Astrophysique et Planétologie, il paraît indispensable
de remercier les personnes qui ont contribué de près ou de loin au déroulement de mon
doctorat.
Je commencerai par Sylvie Roques, qui m’a soutenue et m’a donné la chance de com-
mencer cette thèse. Merci également à Dominique Lullier et Marie-Claude Cathala pour
leur gentillesse et leur efficacité à résoudre mes problèmes administratifs.
Je suis bien entendu reconnaissante à tous les membres de mon jury de thèse : Andrew
Collier Cameron et Jérôme Bouvier qui ont accepté de prendre de leur temps précieux pour
rapporter mon manuscrit, Michel Rieutord qui a présidé ce jury, Arturo López Ariste,
Agnès Lèbre et Boris Dintrans qui ont été des examinateurs très impliqués, ainsi que
Pascal Petit, mon directeur de thèse. Il m’est très difficile d’exprimer mes remerciements
à Pascal en quelques mots. Son mérite le plus grand est sans doute d’avoir réussi la tâche
extrêmement difficile qui incombe à un directeur de thèse : amener progressivement son
étudiant à développer un travail et une réflexion scientifiques autonomes.
Je remercie les membres de l’équipe de Physique du Soleil et des Etoiles de Toulouse et
de Tarbes dans laquelle j’ai évolué, spécialement Jérôme, Boris, Laurène et Michel pour les
discussions et collaborations scientifiques qui m’ont beaucoup apportées. Je mentionnerai
aussi l’équipe du Télescope Bernard Lyot qui a fait de mes nuits au Pic du Midi des
moments mémorables. Merci donc à Yves, Gilles, Simon, Jean-Pierre, Jean-Marie, Pascal
et Eric.
J’ai beaucoup apprécié la dimension cosmopolite des bureaux que j’ai occupés, prin-
cipalement en compagnie de Valérie et Maria Eliana, et plus brièvement Alexandre, Luis
et Dana. Je n’oublie pas non plus les thésards avec qui j’ai passés de très bons moments :
Nicolas, Simon, Mickael, Vincent, Etienne, Rim, Julien, Gaël, Joël, Sylvain, Mélanie, Guil-
hem, Audrey, Céline, Laurianne et Aurélia.
Je tiens à exprimer ma profonde reconnaissance à deux personnes particulières : Sylvie,
qui a su m’écouter et m’encourager chaque fois que j’en avais besoin, et Pierre, avec qui
j’ai beaucoup aimé partager nos points de vue de thésards sur la recherche et sur la vie
en général.
Je remercie ma famille, en particulier mes parents et mon frère, de m’avoir apporté une
aide matérielle et émotionnelle durant ces longues années d’études. Evidemment et enfin,
je dis toute ma gratitude à Joseph, mon mari et meilleur ami, sans lequel rien n’aurait été
possible, et à ma petite Melyane qui est un rayon de soleil permanent depuis son arrivée.
3