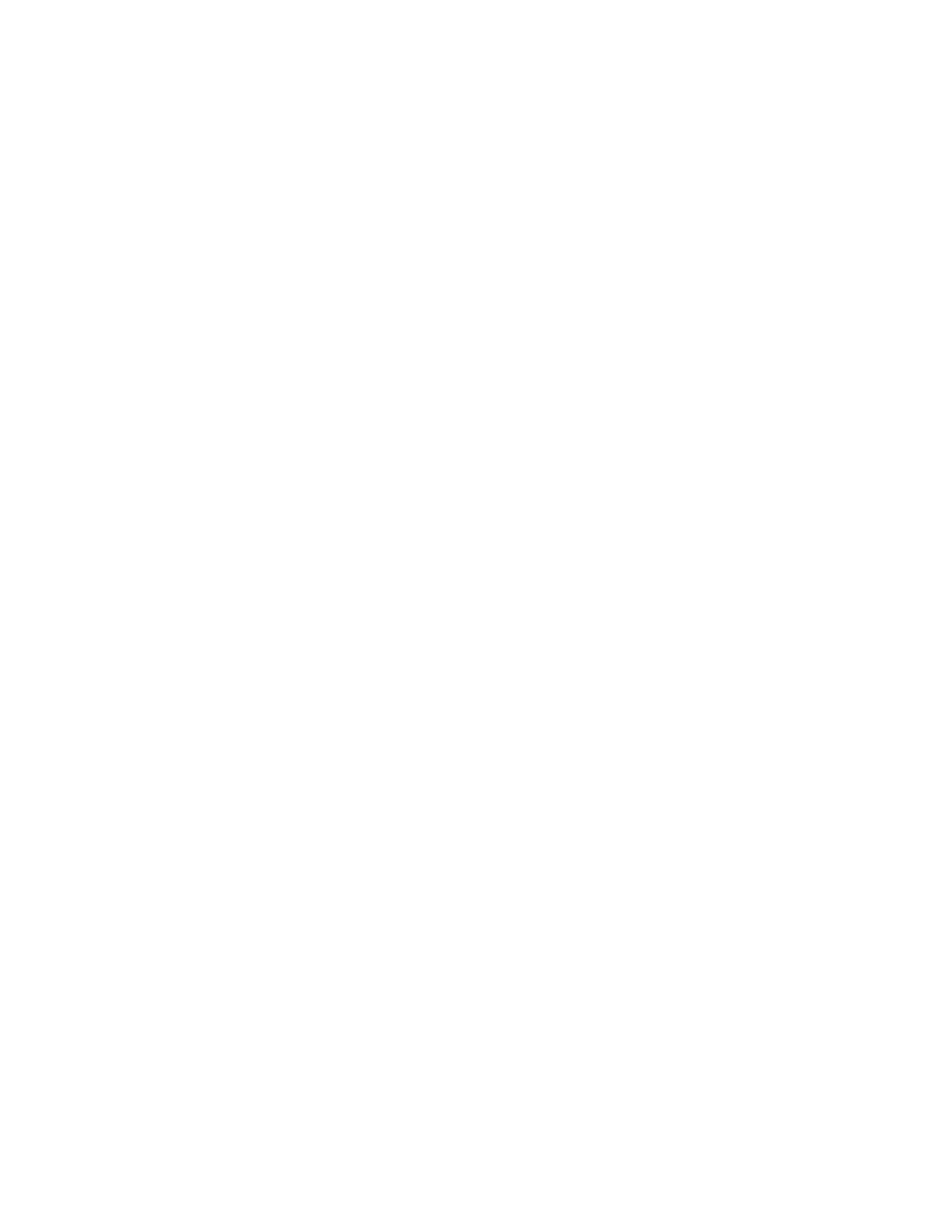
iii
RÉSUMÉ
La plupart des conditions détectées par le dépistage néonatal sont
reliées à l'une des enzymes qui dégradent les acyls-CoA mitochondriaux. Le
rôle physiopathologique des acyls-CoA dans ces maladies est peu connue, en
partie parce que les esters liés au CoA sont intracellulaires et les échantillons
tissulaires de patients humains ne sont généralement pas disponibles. Nous
avons créé une modèle animal murin de l'une de ces maladies, la déficience
en 3-hydroxy-3-methylglutaryl-CoA lyase (HL), dans le foie (souris HLLKO).
HL est la dernière enzyme de la cétogenèse et de la dégradation de la
leucine. Une déficience chronique en HL et les crises métaboliques aigües,
produisent chacune un portrait anormal et distinct d'acyls-CoA hépatiques.
Ces profils ne sont pas prévisibles à partir des niveaux d'acides organiques
urinaires et d'acylcarnitines plasmatiques. La cétogenèse est indétectable
dans les hépatocytes HLLKO. Dans les mitochondries HLLKO isolées, le
dégagement de 14CO2 à partir du [2-14C]pyruvate a diminué en présence de 2-
ketoisocaproate (KIC), un métabolite de la leucine. Au test de tolérance au
pyruvate, une mesure de la gluconéogenèse, les souris HLLKO ne présentent
pas la réponse hyperglycémique normale. L'hyperammoniémie et
l'hypoglycémie, des signes classiques de plusieurs erreurs innées du
métabolisme (EIM) des acyls-CoA, surviennent de façon spontanée chez des
souris HLLKO et sont inductibles par l'administration de KIC. Une charge en
KIC augmente le niveau d'acyls-CoA reliés à la leucine et diminue le niveau
d'acétyl-CoA. Les mitochondries des hépatocytes des souris HLLKO traitées