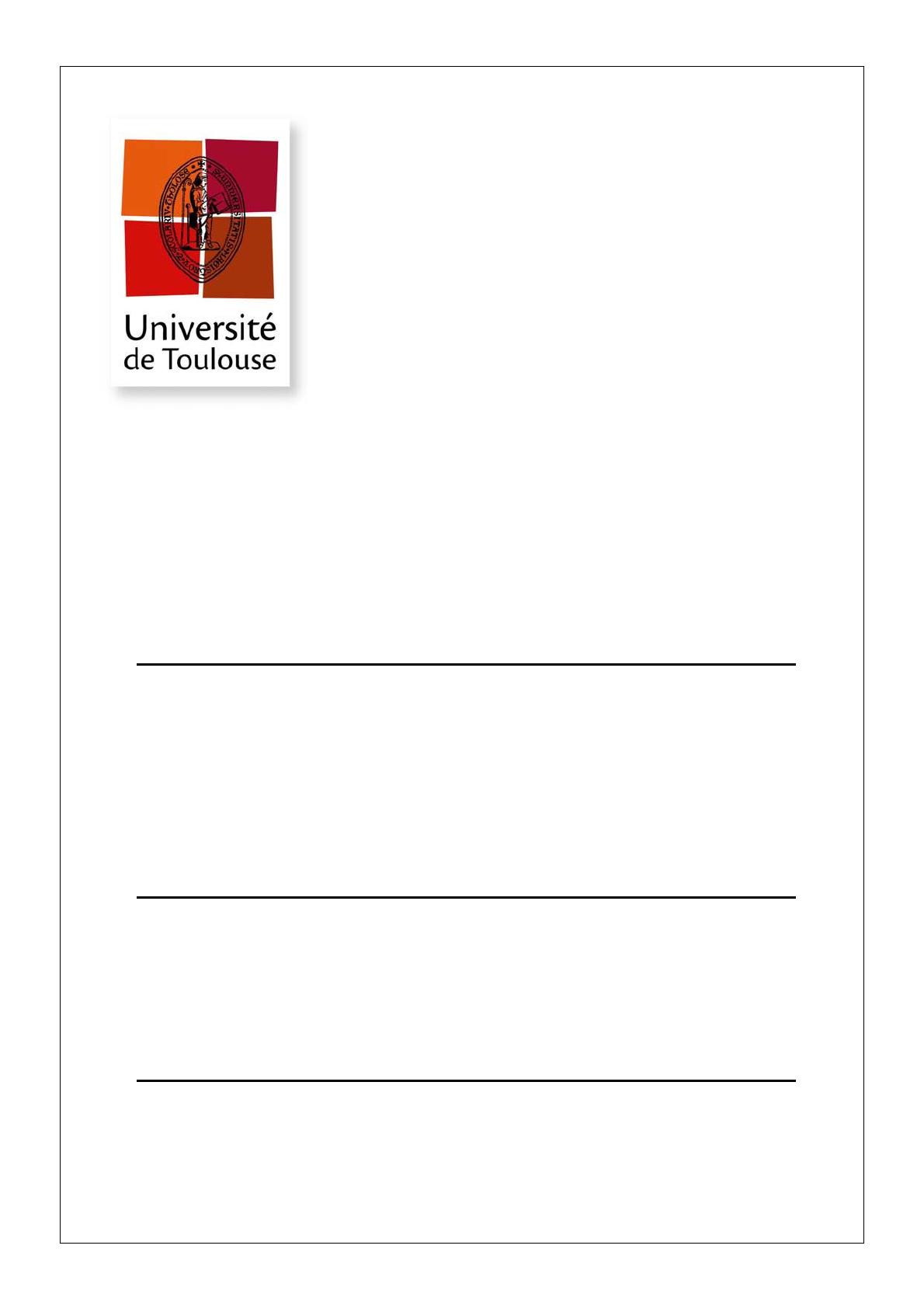
T
TH
HÈ
ÈS
SE
E
En vue de l'obtention du
D
DO
OC
CT
TO
OR
RA
AT
TD
DE
EL
L’
’U
UN
NI
IV
VE
ER
RS
SI
IT
TÉ
ÉD
DE
ET
TO
OU
UL
LO
OU
US
SE
E
Délivré par l'Université Toulouse III - Paul Sabatier
Discipline ou spécialité : Géophysique
JURY
Claude Jaupart Président Institut de Physique du Globe de Paris
Edouard Kaminski Rapporteur Institut de Physique du Globe de Paris
Jean François Lénat Rapporteur Université Blaise Pascal Clermont II
Lionel D’USTON Examinateur CESR - Université Toulouse III
Kei Kurita Examinateur ERI - Université de Tokyo
Ecole doctorale : Sciences de l’Univers, de l’Environnement et de l’Espace
Unité de recherche : Laboratoire Dynamique Terrestre et Planétaire
Directeur(s) de Thèse : Michel Rabinowicz, David Baratoux et Patrick Bachèlery
Présentée et soutenue par Raphaël ANTOINE
Le
Exploration et modélisation de la circulation
d’air dans le Piton de la Fournaise
et Cerberus Fossae (Mars)
10 Juillet 2009