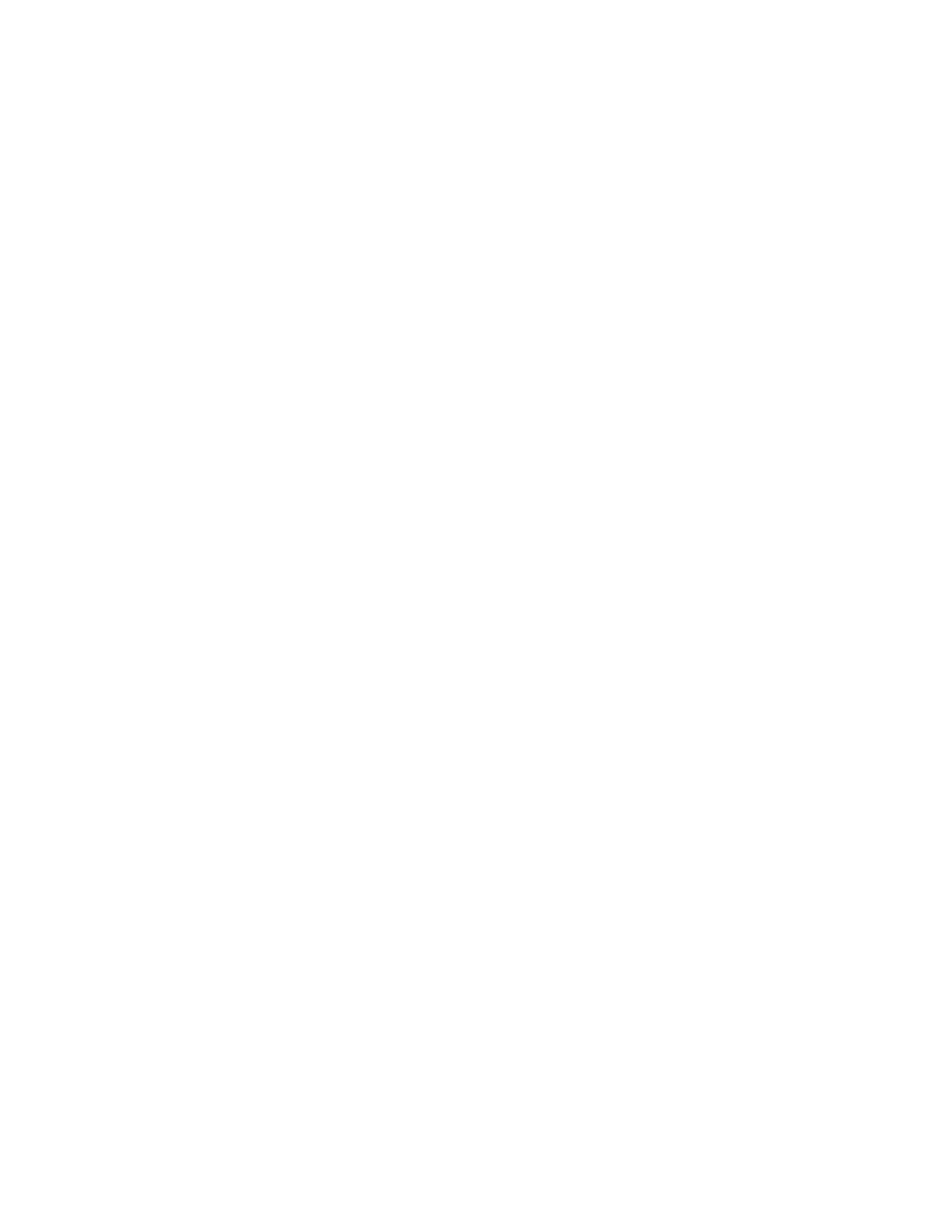
i
RÉSUMÉ
La dépression est une pathologie grave qui, malgré de multiples stratégies
thérapeutiques, demeure résistante chez un tiers des patients. Les techniques de stimulation
cérébrale sont devenues une alternative intéressante pour les patients résistants à diverses
pharmacothérapies. La stimulation du nerf vague (SNV) a ainsi fait preuve de son
efficacité en clinique et a récemment été approuvée comme traitement additif pour la
dépression résistante. Cependant, les mécanismes d’action de la SNV en rapport avec la
dépression n’ont été que peu étudiés.
Cette thèse a donc eu comme premier objectif de caractériser l’impact de la SNV
sur les différents systèmes monoaminergiques impliqués dans la pathophysiologie de la
dépression, à savoir la sérotonine (5-HT), la noradrénaline (NA) et la dopamine (DA),
grâce à l’utilisation de techniques électrophysiologiques et de la microdialyse in vivo chez
le rat. Des études précliniques avaient déjà révélé qu’une heure de SNV augmente le taux
de décharge des neurones NA du locus coeruleus, et que 14 jours de stimulation sont
nécessaires pour observer un effet comparable sur les neurones 5-HT. Notre travail a
démontré que la SNV modifie aussi le mode de décharge des neurones NA qui présente
davantage de bouffées, influençant ainsi la libération terminale de NA, qui est
significativement augmentée dans le cortex préfrontal et l’hippocampe après 14 jours.
L’augmentation de la neurotransmission NA s’est également manifestée par une élévation
de l’activation tonique des récepteurs postsynaptiques α2-adrénergiques de l’hippocampe.
Après lésion des neurones NA, nous avons montré que l’effet de la SNV sur les neurones
5-HT était indirect, et médié par le système NA, via l’activation des récepteurs α1-
adrénergiques présents sur les neurones du raphé. Aussi, tel que les antidépresseurs
classiques, la SNV augmente l’activation tonique des hétérorécepteurs pyramidaux 5-