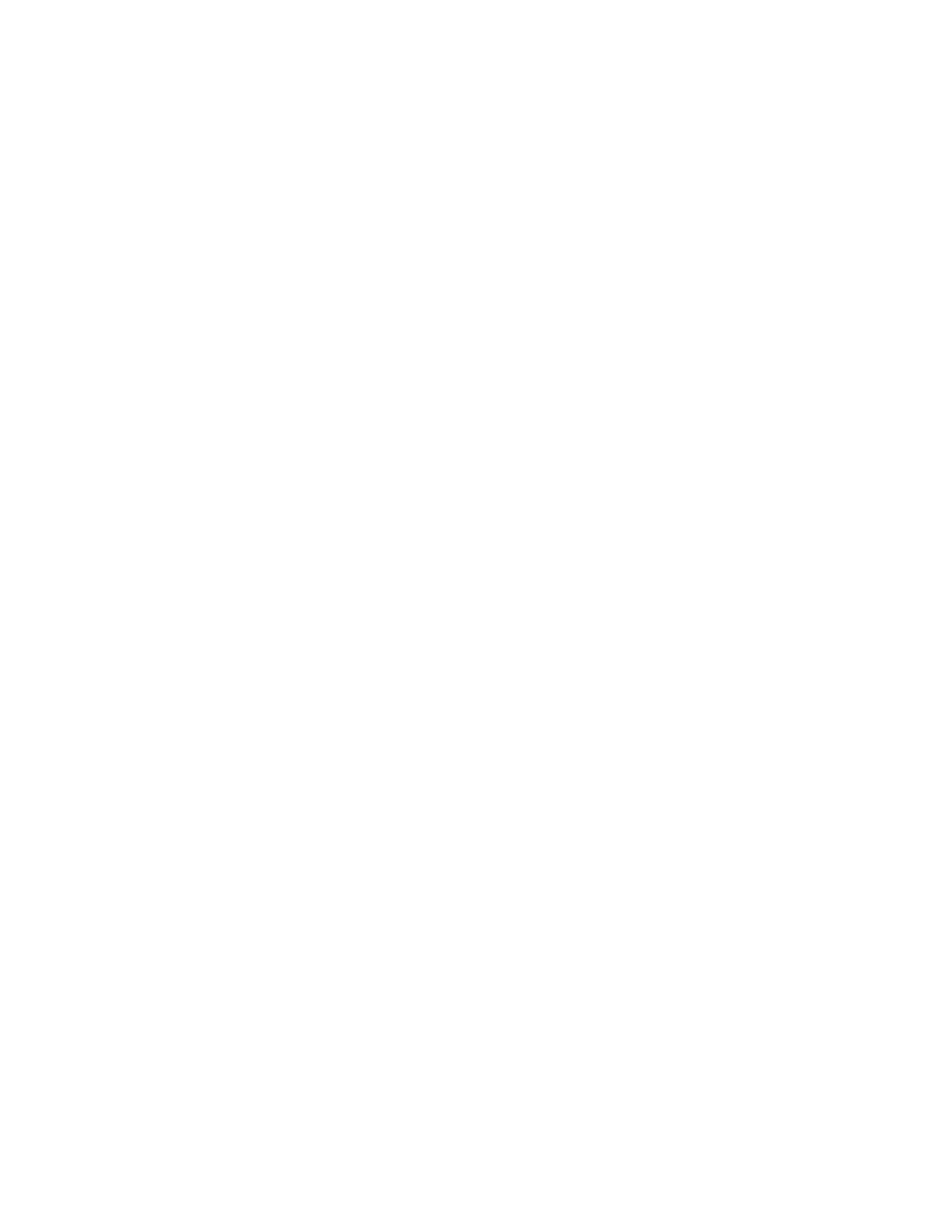
iv
REMERCIEMENTS
Mes sincères et amicales remerciements à Sébastien Rifflart (Total), sans qui ce projet ne
serait demeuré qu’un projet de recherche de quelques mois. Grâce à sa vision des retombées
potentielles des résultats préliminaires de ce premier projet de recherche, une maîtrise, un
brevet et une thèse sont nés. Je remercie également et sincèrement Hélène Stainton et Mahdi
Yazdapanah de chez Total avec qui j’ai eu des échanges très constructifs.
Merci à mes collègues, en particulier à François-Xavier Chiron, qui a su m’amener à des
niveaux supérieurs en pointant mes lacunes et possibilités. Au plaisir de te croiser dans une
conférence et d’en boire quelques-unes à ta santé.
Merci au professeur émérite Michel Soustelle, de l’École nationale supérieure des mines de
Saint-Étienne, avec qui j’ai échangé et qui m’a grandement éclairé sur les voies de modélisation
des réactions gaz-solide en tenant compte des caractéristiques des solides réels.
Je tiens à remercier les professeurs du département de Génie chimique, en particulier au
chargé d’enseignement Patrice Farand, pour les nombreuses possibilités d’enseignement, ainsi
que Jamal Chaouki et Robert Legros pour m’avoir montré de la considération. Merci aussi aux
techniciens, en particulier Jean Huard qui doit se réveiller en sursaut la nuit en m’entendant
lui demander des raccords Swagelok...
Je ne peux également passer sous silence la grande source d’inspiration que représente Marisol
López Vera, ma femme. Elle a entièrement appuyé mon retour aux études après quelques
années de travail en industrie, malgré la baisse de salaire qui en résultait. C’est elle qui
a tenu le fort familial durant certaines soirées et fins de semaine d’expérimentation et de
rédaction de thèse. Elle a aussi été le ciment familial et de couple. Je te remercie du fond
de mon coeur. Merci aussi à Mateo, mon grand de 6 ans, une source d’inspiration : je me
souviendrai toute ma vie de cette fois où tu t’es assis devant mon ordi en me disant que tu
travaillais sur ton doctorat ! Merci aussi à Nathan, mon bébé naissant, qui me permet de
réfléchir à des questions complexes de modélisation en ne dormant pas la nuit ! Merci ma
petite famille.
Merci également à Miles Davis, John Coltrane, Lila Downs, Conchita Buika, Bernard Ada-
mus, René Aubry et Tom Waits (entre autres) de m’avoir accompagné durant le long travail
de traitement de données, de modélisation et de rédaction.
Finalement, je tiens à remercier Gregory-S. Patience, mon directeur de thèse, sans qui je
serais probablement encore un ingénieur non gradué oeuvrant à des tâches répétitives. Par