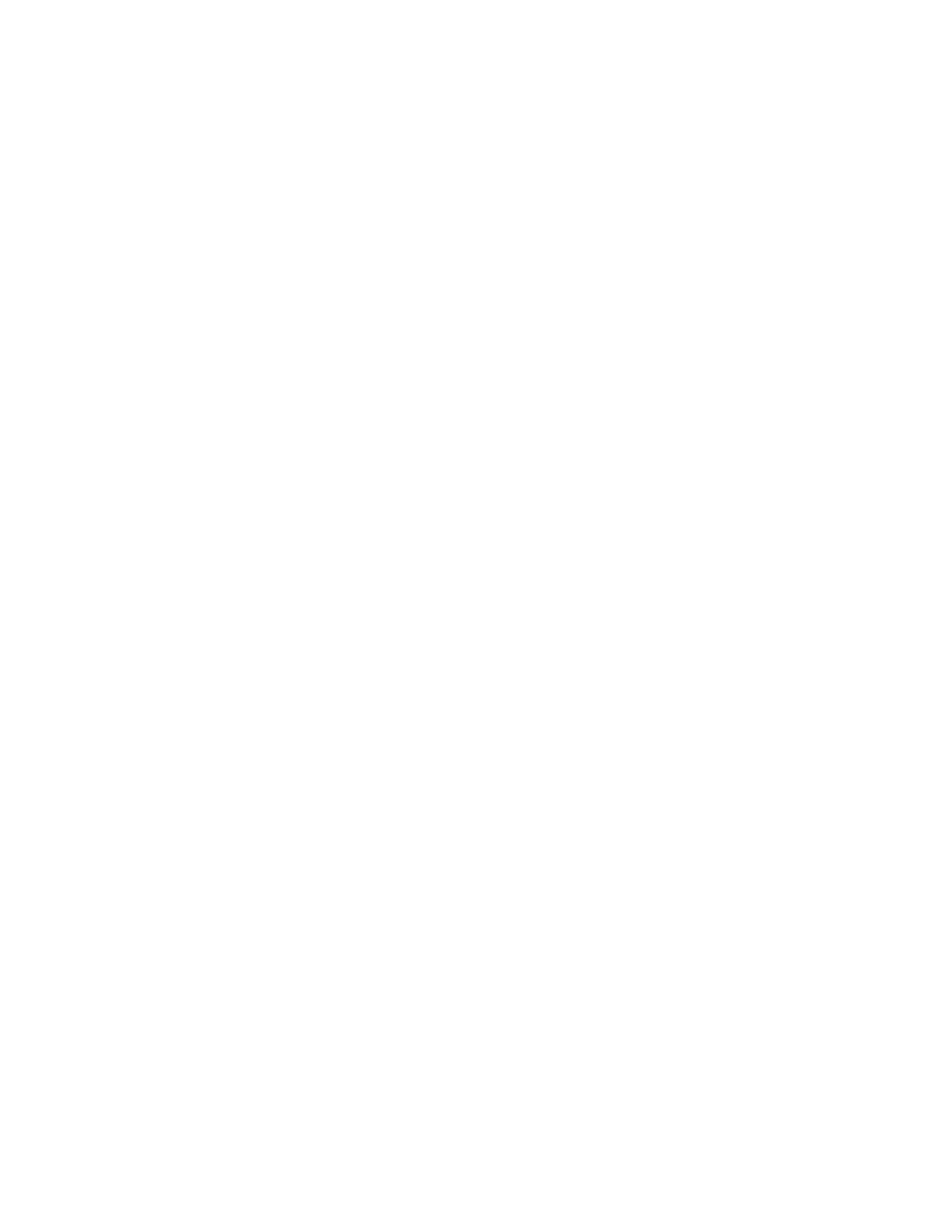
Acknowledgements
I would like to express my sincere gratitude to my supervisor Dr. Jürgen Gailer for giving
me the opportunity to work on these fascinating projects. His perpetual enthusiasm,
encouragement, and guidance have inspired me to broaden my knowledge and strengthen my
analytical skills.
I owe my deepest gratitude to Dr. Aru Narendran for his assistance and advice, as well as
for his help with the recruitment of cancer patients. I would also like to thank Karen Mazil for
her constant assistance with the ethics submission for this project, as well as with the recruitment
of the cancer patients.
I wish to express my warm and sincere thanks to Prof. Kevin Thurbide for being in my
supervisory committee and for all of the analytical knowledge he passed on. I am very grateful to
Dr. Roland Roesler for serving on my supervisory committee. It is a pleasure for me to thank Dr.
Ann-Lise Norman for accepting to be the internal examiner, and to Dr. Thomas Baumgartner for
being the neutral chair on my defense committee.
I am grateful to Mandy Chan at the Canadian Sport Centre, University of Calgary for
being so helpful in drawing blood from my volunteers for research. I would like to thank all of
the volunteers for being so generous to donate blood for my research.
Many thanks go to the Department of Chemistry administrative staff, especially to
Bonnie King and Janice Crawford for being so kind in clarifying all my questions. Alberta
Innovates Health Solution is greatly acknowledged for their financial support for my project, as
well as the University of Calgary for their financial support during my degree period.
It was a great pleasure to work with the past and current members of the Gailer group. I
would like to thank Melani and Elham for all of their help, advice, and support they provided