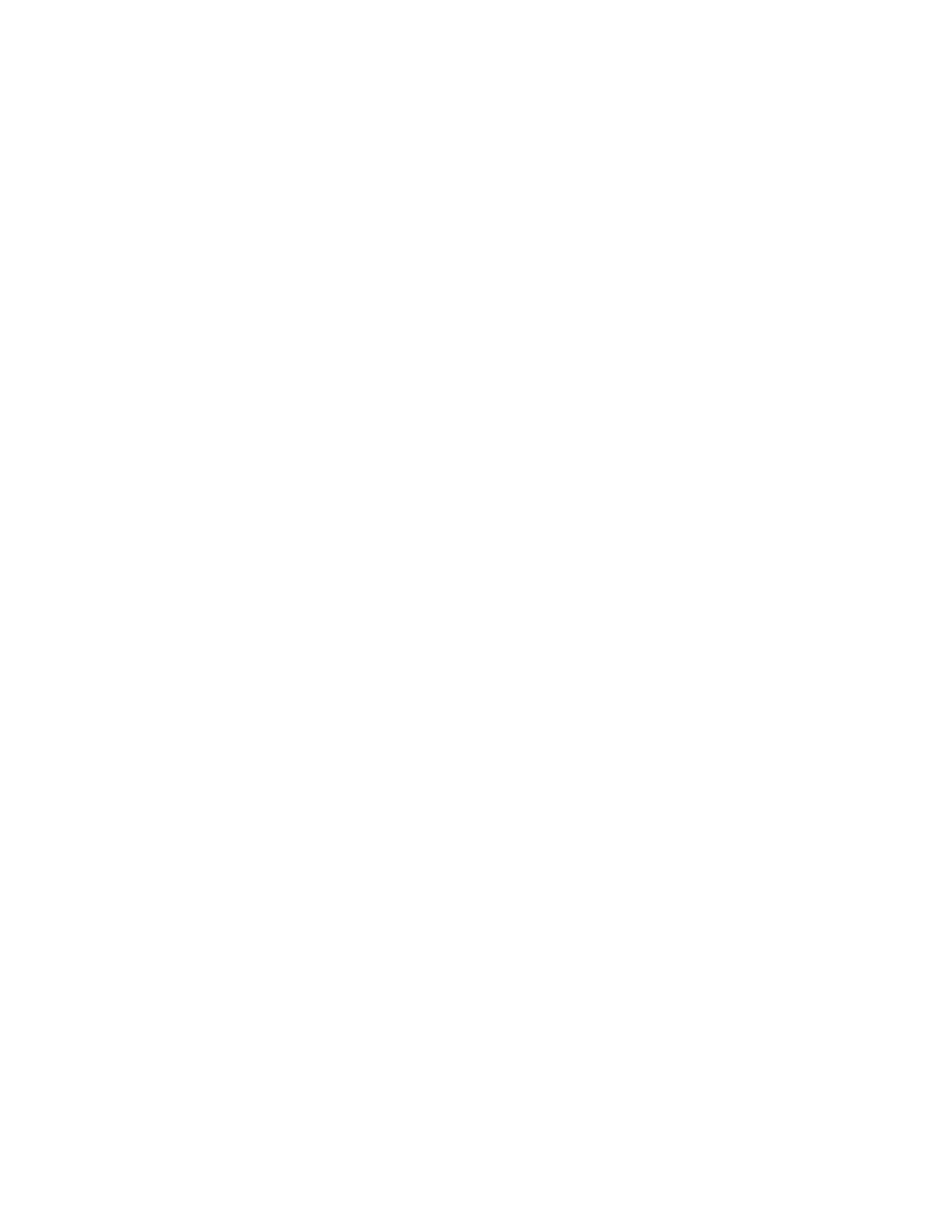
Discovery and Mass Measurements of a Cold, 10-Earth Mass Planet and Its
Host Star
Y. Muraki1,67, C. Han2,68,∗, D.P. Bennett3,67,70,∗, D. Suzuki4,67, L.A.G. Monard5,68,
R. Street6,69, U.G. Jorgensen7,71, P. Kundurthy8, J. Skowron9,68, A.C. Becker8,
M.D. Albrow10,70, P. Fouqu´e11,70, D. Heyrovsk´y12, R.K. Barry13, J.-P. Beaulieu14,70,
D.D. Wellnitz15, I.A. Bond16,67, T. Sumi4,17,67, S. Dong18,68, B.S. Gaudi9,68,
D.M. Bramich19,69, M. Dominik20,21,69,71,
and
F. Abe4, C.S. Botzler22, M. Freeman22, A. Fukui4, K. Furusawa4, F. Hayashi4,
J.B. Hearnshaw10, S. Hosaka4, Y. Itow4, K. Kamiya4, A.V. Korpela23, P.M. Kilmartin24,
W. Lin16, C.H. Ling16, S. Makita4, K. Masuda4, Y. Matsubara4, N. Miyake4, K. Nishimoto4,
K. Ohnishi25, Y.C. Perrott22, N.J. Rattenbury26, To. Saito27, L. Skuljan16,
D.J. Sullivan23, W.L. Sweatman16, P.J. Tristram24, K. Wada1, P.C.M. Yock22,
(The MOA Collaboration)
G.W. Christie28, D.L. DePoy29, E. Gorbikov30, A. Gould9, S. Kaspi30, C.-U. Lee31,
F. Mallia32, D. Maoz30, J. McCormick33, D. Moorhouse34, T. Natusch28, B.-G. Park31,
R.W. Pogge9, D. Polishook35, A. Shporer30, G. Thornley34, J.C. Yee9,
(The µFUN Collaboration)
A. Allan36, P. Browne20,71, K. Horne20, N. Kains19,
C. Snodgrass37,38,71, I. Steele39, Y. Tsapras6,40,
(The RoboNet Collaboration)
V. Batista14, C.S. Bennett41, S. Brillant37, J.A.R. Caldwell42, A. Cassan14, A. Cole43,
R. Corrales14, Ch. Coutures14, S. Dieters43, D. Dominis Prester44, J. Donatowicz45,
J. Greenhill43, D. Kubas14,37, J.-B. Marquette14, R. Martin46, J Menzies47,
K.C. Sahu48, I. Waldman49, A. Williams46 M. Zub50,
(The PLANET Collaboration)
H. Bourhrous51, Y. Matsuoka52, T. Nagayama52, N. Oi53, Z. Randriamanakoto47,
(IRSF Observers)
V. Bozza54,55, M.J. Burgdorf56,57, S. Calchi Novati54, S. Dreizler58, F. Finet59, M. Glitrup6,
K. Harpsøe7, T.C. Hinse7,31, M. Hundertmark58, C. Liebig20, G. Maier50,
L. Mancini54,61, M. Mathiasen7, S. Rahvar62, D. Ricci59, G. Scarpetta54,55, J. Skottfelt7,
J. Surdej59, J. Southworth63, J. Wambsganss50, F. Zimmer50,
(The MiNDSTEp Consortium)
A. Udalski64, R. Poleski64, L. Wyrzykowski64,65, K. Ulaczyk64, M.K. Szyma´nski64,
M. Kubiak64, G. Pietrzy´nski64,66, I. Soszy´nski64
(The OGLE Collaboration)
arXiv:1106.2160v1 [astro-ph.EP] 10 Jun 2011