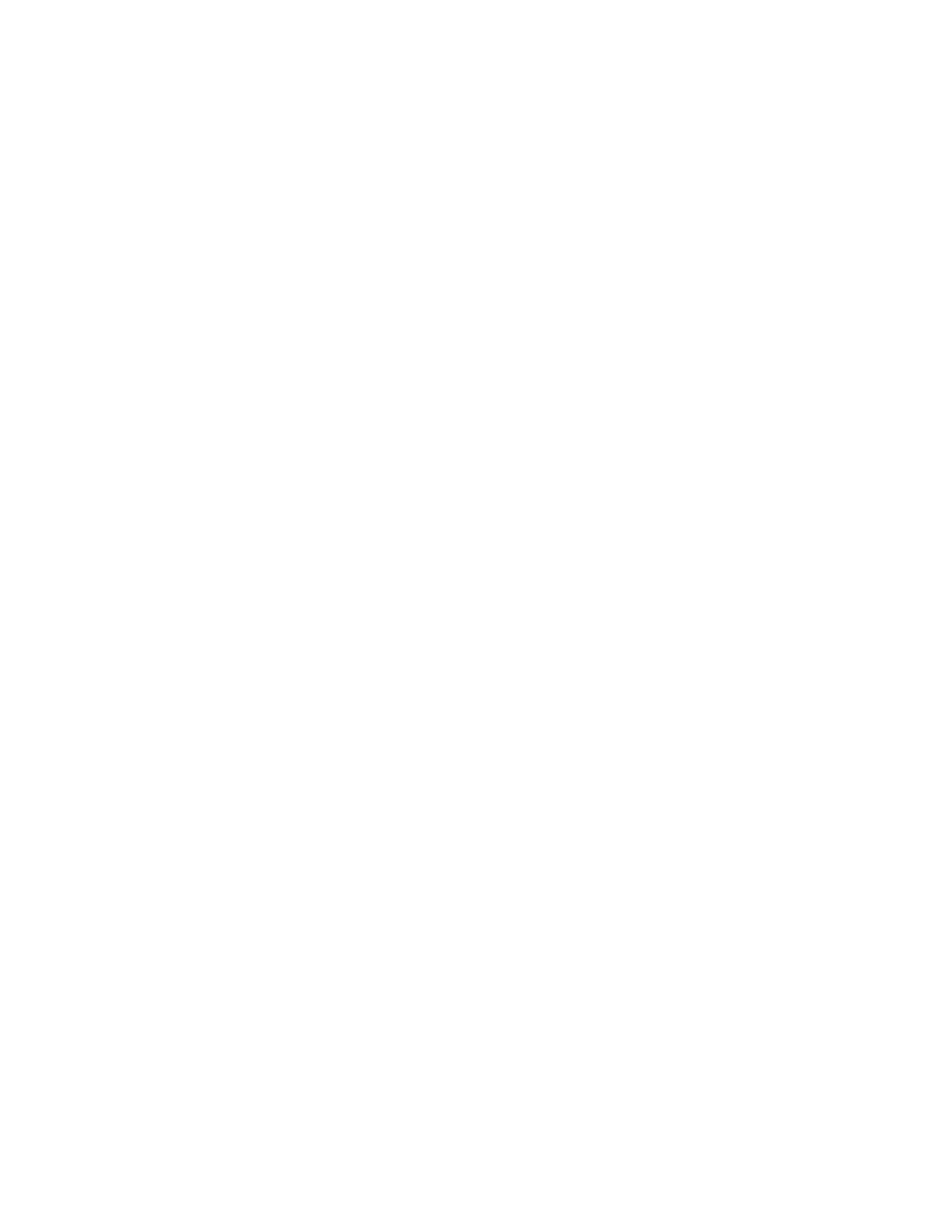
arXiv:1309.7714v2 [astro-ph.EP] 10 Oct 2013
MOA-2010-BLG-328Lb: a sub-Neptune orbiting very late M
dwarf?
K. Furusawa1,A, A. Udalski2,B , T. Sumi3,1,A, D.P. Bennett4,A,C , I.A. Bond5,A, A. Gould6,D,
U.G. Jørgensen7,8,E , C. Snodgrass9,10,F,E, D. Dominis Prester11,C , M.D. Albrow12,C ,
and
F. Abe1, C.S. Botzler13, P. Chote14, M. Freeman13, A. Fukui15, P. Harris14, Y. Itow1,
C.H. Ling5, K. Masuda1, Y. Matsubara1, N. Miyake1, Y. Muraki1, K. Ohnishi16,
N.J. Rattenbury13, To. Saito17, D.J. Sullivan14, D. Suzuki3, W.L. Sweatman5,
P.J. Tristram18, K. Wada3, P.C.M. Yock13,
(The MOA Collaboration)
M.K. Szyma´nski2, I. Soszy´nski2, M. Kubiak2, R. Poleski2, K. Ulaczyk2, G. Pietrzy´nski2,19,
L. Wyrzykowski2,20,
(The OGLE Collaboration)
J.-Y. Choi21, G.W. Christie22, D.L. DePoy23, Subo Dong24, J. Drummond25, B.S. Gaudi6,
C. Han21, L.-W. Hung26,6, K.-H. Hwang21, C.-U. Lee27, J. McCormick28, D. Moorhouse29,
T. Natusch22,30, M. Nola29, E. Ofek31, R.W. Pogge6, I.-G. Shin21, J. Skowron6,
G. Thornley29, J.C. Yee6,
(The µFUN Collaboration)
K.A. Alsubai32, V. Bozza33,34, P. Browne35,F , M.J. Burgdorf36, S. Calchi Novati33,37,
P. Dodds35, M. Dominik35,38,C,F , F. Finet39, T. Gerner40, S. Hardis7, K. Harpsøe7,8,
T.C. Hinse7,41,27, M. Hundertmark35,42, N. Kains43,35,F , E. Kerins44, C. Liebig35,40,
L. Mancini33,45, M. Mathiasen7, M.T. Penny6,44, S. Proft40, S. Rahvar46,47, D. Ricci39,
G. Scarpetta33,34, S. Sch¨afer42, F. Sch¨onebeck40, J. Southworth48, J. Surdej42,
J. Wambsganss40,
(The MiNDSTEp Consortium)
R.A. Street49, D.M. Bramich43, I.A. Steele50, Y. Tsapras49,51,
(The RoboNet Collaboration)
K. Horne35,F , J. Donatowicz52, K.C. Sahu53,E , E. Bachelet54, V. Batista6,55, T.G. Beatty6,
J.-P. Beaulieu55, C.S. Bennett56, C. Black57, R. Bowens-Rubin58, S. Brillant10,
J.A.R. Caldwell59, A. Cassan55, A.A. Cole57, E. Corrales55, C. Coutures55, S. Dieters57,
P. Fouqu´e54, J. Greenhill57, C.B. Henderson6, D. Kubas10,55, J.-B. Marquette55,
R. Martin60, J.W. Menzies61, B. Shappee6, A. Williams60, D. Wouters62, J. van Saders6,
R. Zellem63, M. Zub39
(The PLANET Collaboration)