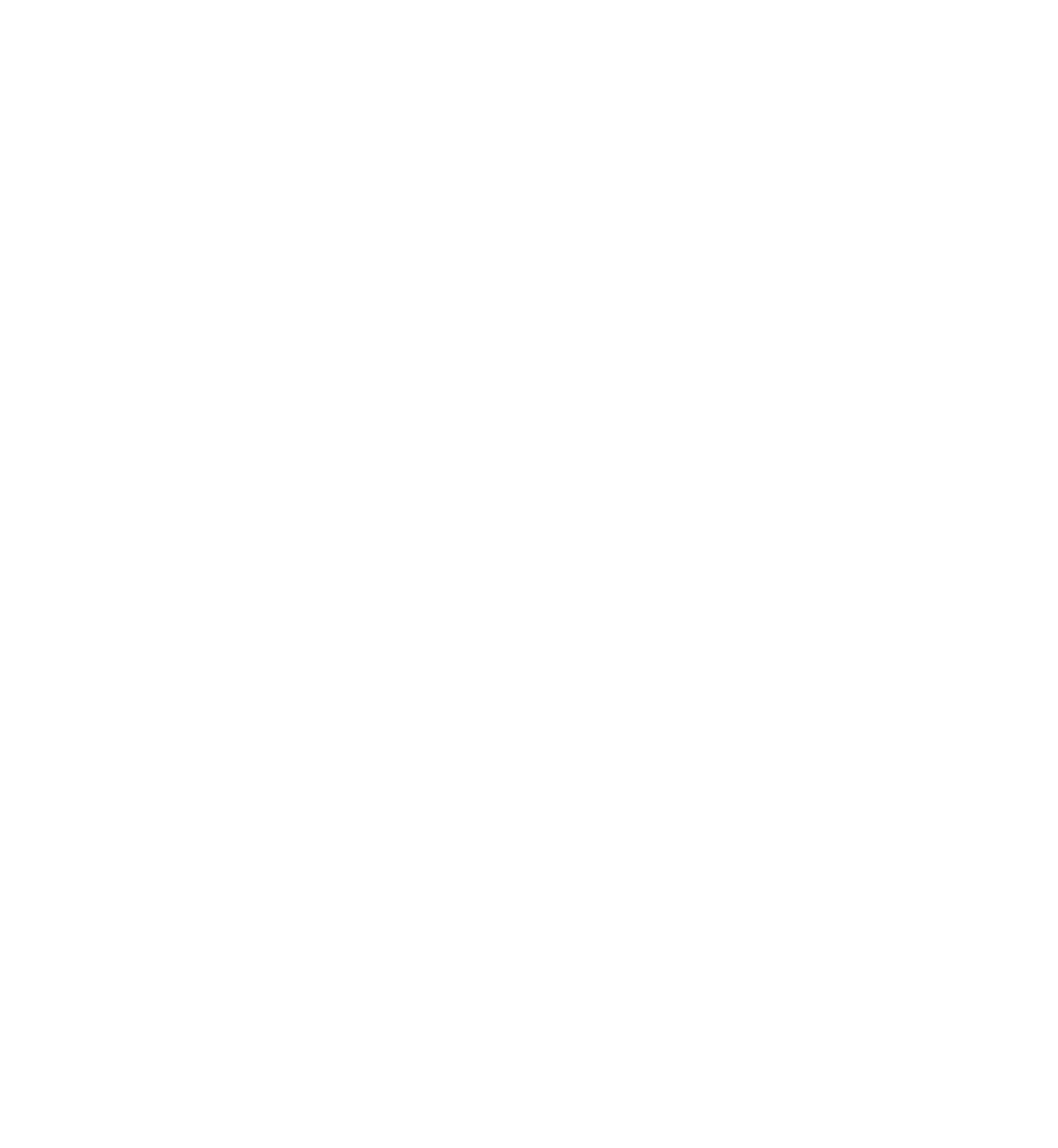
iv
que sur de courtes distances. Par conséquent, ils ne sont pas adaptés pour l‘examen de l‘artère fémorale, à cause de sa
longueur et de sa forme tortueuse.
L‘intérêt pour la robotique médicale date des années 70. Depuis, plusieurs robots médicaux ont été proposés
pour la chirurgie, la thérapie et le diagnostic. Dans le cas du diagnostic artériel, seuls deux prototypes sont proposés,
mais non commercialisés. Hippocrate est le premier robot de type maitre/esclave conçu pour des examens des petits
segments d‘artères (carotide). Il est composé d‘un bras à 6 degrés de liberté (ddl) suspendu au-dessus du patient sur un
socle rigide. À partir de ce prototype, un contrôleur automatisant les déplacements du robot par rétroaction des images
échographiques a été conçu et testé sur des fantômes. Le deuxième est le robot de la Colombie Britannique conçu pour
les examens à distance de la carotide. Le mouvement de la sonde est asservi par rétroaction des images US. Les
travaux publiés avec les deux robots se limitent à la carotide.
Afin d‘examiner un long segment d‘artère, un système robotique US a été conçu dans notre laboratoire. Le
système possède deux modes de fonctionnement, le mode teach/replay (voir annexe 2) et le mode commande libre par
l‘utilisateur. Dans ce dernier mode, l‘utilisateur peut implémenter des programmes personnalisés comme ceux utilisés
dans ce projet afin de contrôler les mouvements du robot.
Le but de ce projet est de démontrer les performances de ce système robotique dans des conditions proches au
contexte clinique avec le mode commande libre par l‘utilisateur. Deux objectifs étaient visés: (1) évaluer in vitro le
suivi automatique et la reconstruction 3D en temps réel d‘une artère en utilisant trois fantômes ayant des géométries
réalistes. (2) évaluer in vivo la capacité de ce système d'imagerie robotique pour la cartographie 3D en temps réel d'une
artère fémorale normale. Pour le premier objectif, la reconstruction 3D US a été comparée avec les fichiers CAD
(computer-aided-design) des fantômes. De plus, pour le troisième fantôme, la reconstruction 3D US a été comparée
avec sa reconstruction CTA, considéré comme examen de référence pour évaluer la MAP.
Cinq chapitres composent ce mémoire. Dans le premier chapitre, la MAP sera expliquée, puis dans les deuxième et
troisième chapitres, l‘imagerie 3D ultrasonore et la robotique médicale seront développées. Le quatrième chapitre sera
consacré à la présentation d‘un article intitulé " A robotic ultrasound scanner for automatic vessel tracking and
three-dimensional reconstruction of B-mode images" qui résume les résultats obtenus dans ce projet de maîtrise. Une
discussion générale conclura ce mémoire.
L‘article intitulé " A 3D ultrasound imaging robotic system to detect and quantify lower limb arterial
stenoses: in vivo feasibility " de Marie-Ange Janvier et al dans l‘annexe 2, permettra également au lecteur de mieux