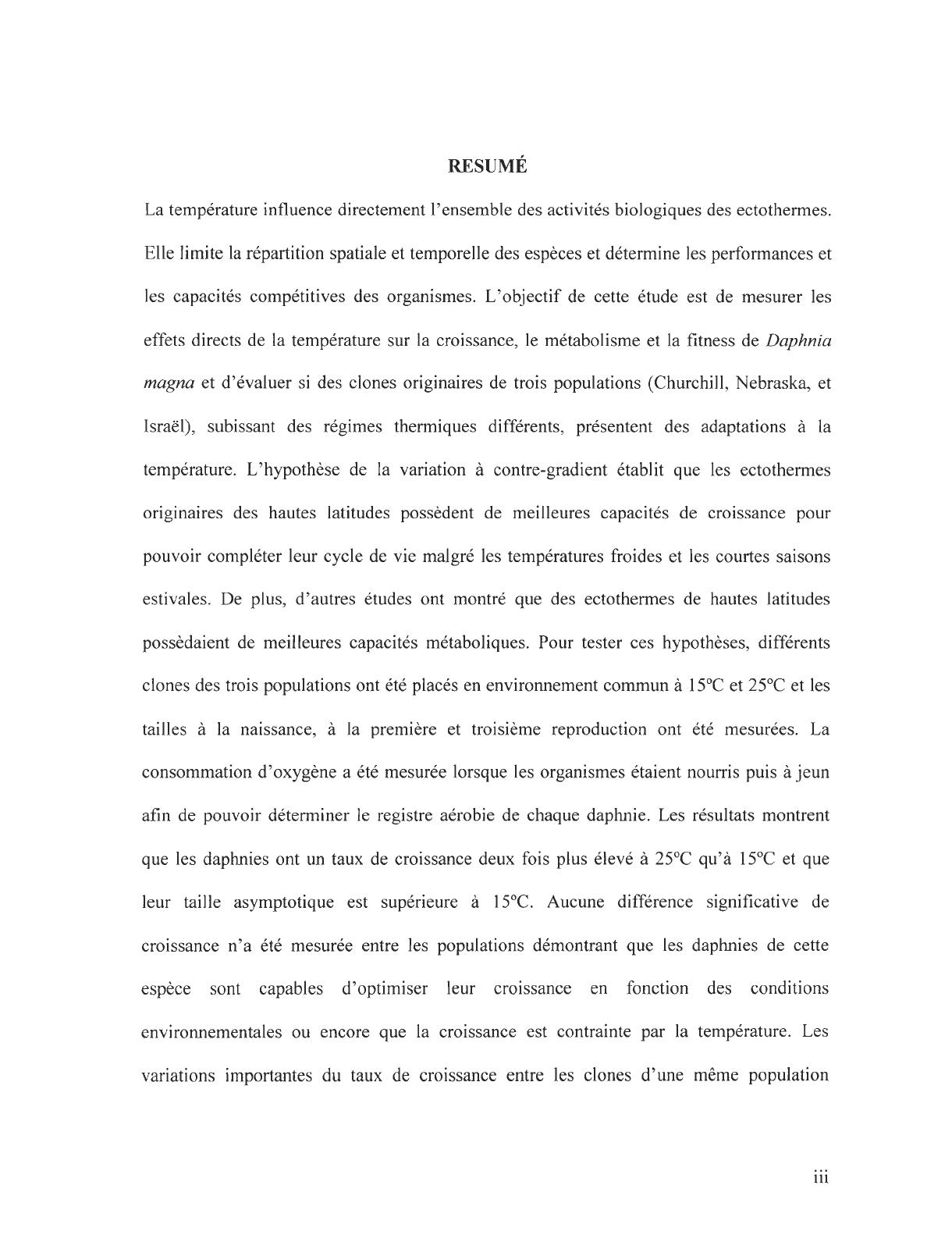
RESUMÉ
La température influence directement l'ensemble des activités biologiques des ectothermes.
Elle limite
la
répartition spatiale et temporelle des espèces et détermine les performances et
les capacités compétitives des organismes. L'objectif de cette étude est de mesurer les
effets directs de la température sur la croissance,
le
métabolisme et la fitness de Daphnia
magna et d'évaluer
si
des clones originaires de trois populations (Churchill, Nebraska, et
Israël), subissant des régimes thermiques différents, présentent des adaptations à la
température. L'hypothèse
de
la variation à contre-gradient établit que les ectothermes
originaires des hautes latitudes possèdent de meilleures capacités de croissance pour
pouvoir compléter leur cycle de vie malgré les températures froides et les courtes saisons
estivales.
De
plus, d'autres études ont montré que des ectothermes de hautes latitudes
possèdaient de meilleures capacités métaboliques. Pour tester ces hypothèses, différents
clones des trois populations ont été placés en environnement commun à
15°C
et 25°C et les
tailles à la naissance, à la première et troisième reproduction ont été mesurées. La
consommation d'oxygène a été mesurée lorsque les organismes étaient nourris puis à jeun
afin de pouvoir déterminer le registre aérobie de chaque daphnie. Les résultats montrent
que les daphnies ont
un
taux de croissance deux fois plus élevé à 25°C qu'à
15
°C et que
leur taille asymptotique est supérieure à
15°C.
Aucune différence significative de
croissance n'a été mesurée entre les populations démontrant que les daphnies de cette
espèce sont capables d'optimiser leur croissance en fonction des conditions
environnementales ou encore que la croIssance est contrainte par la température. Les
variations importantes du taux de croissance entre les clones d'une même population
III