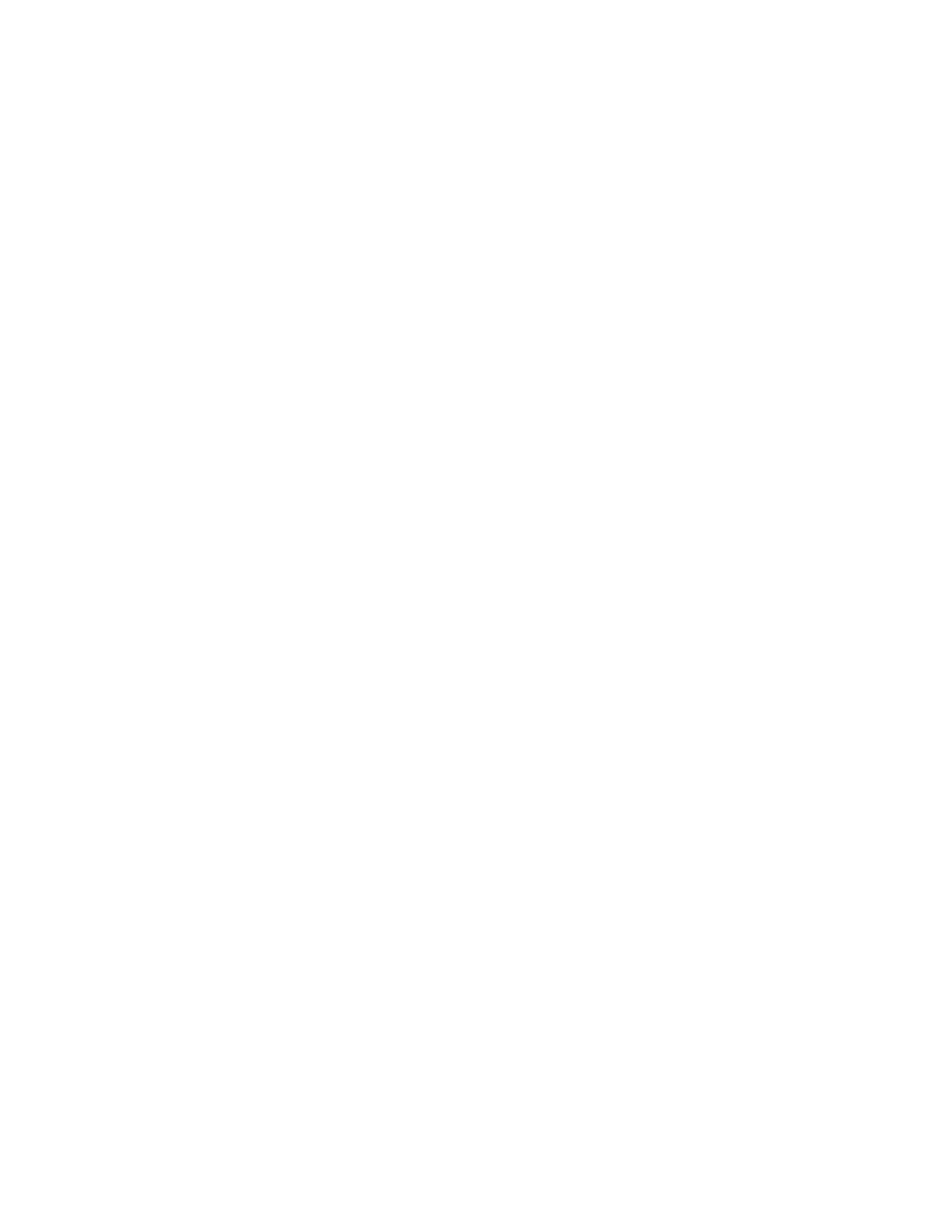
iii
Résumé
Les autotransporteurs monomériques, appartenant au système de sécrétion de type V,
correspondent à une famille importante de facteurs de virulence bactériens. Plusieurs
fonctions, souvent essentielles pour le développement d’une infection ou pour le maintien
et la survie des bactéries dans l’organisme hôte, ont été décrites pour cette famille de
protéines. Malgré l’importance de ces protéines, notre connaissance de leur biogenèse et de
leur mécanisme d’action demeure relativement limitée.
L’autotransporteur AIDA-I, retrouvé chez diverses souches d’Escherichia coli, est un
autotransporter multifonctionnel typique impliqué dans l’adhésion et l’invasion cellulaire
ainsi que dans la formation de biofilm et d’agrégats bactériens. Les domaines
extracellulaires d’autotransporteurs monomériques sont responsables de la fonctionnalité et
possèdent pratiquement tous une structure caractéristique d’hélice β. Nous avons mené une
étude de mutagenèse aléatoire avec AIDA-I afin de comprendre la base de la
multifonctionnalité de cette protéine. Par cette approche, nous avons démontré que les
domaines passagers de certains autotransporteurs possèdent une organisation modulaire, ce
qui signifie qu’ils sont construits sous la forme de modules fonctionnels.
Les domaines passagers d’autotransporteurs peuvent être clivés et relâchés dans le milieu
extracellulaire. Toutefois, malgré la diversité des mécanismes de clivage existants,
plusieurs protéines, telles qu’AIDA-I, sont clivées par un mécanisme qui demeure inconnu.
En effectuant une renaturation in vitro d’AIDA-I, couplée avec une approche de
mutagenèse dirigée, nous avons démontré que cette protéine se clive par un mécanisme
autocatalytique qui implique deux acides aminés possédant un groupement carboxyle. Ces
résultats ont permis la description d’un nouveau mécanisme de clivage pour la famille des
autotransporteurs monomériques.
Une des particularités d’AIDA-I est sa glycosylation par une heptosyltransférase spécifique
nommée Aah. La glycosylation est un concept plutôt récent chez les bactéries et pour
l’instant, très peu de protéines ont été décrites comme glycosylées chez E. coli. Nous avons
démontré que Aah est le prototype pour une nouvelle famille de glycosyltransférases
bactériennes retrouvées chez diverses espèces de protéobactéries. La glycosylation
d’AIDA-I est une modification cytoplasmique et post-traductionnelle. De plus, Aah ne