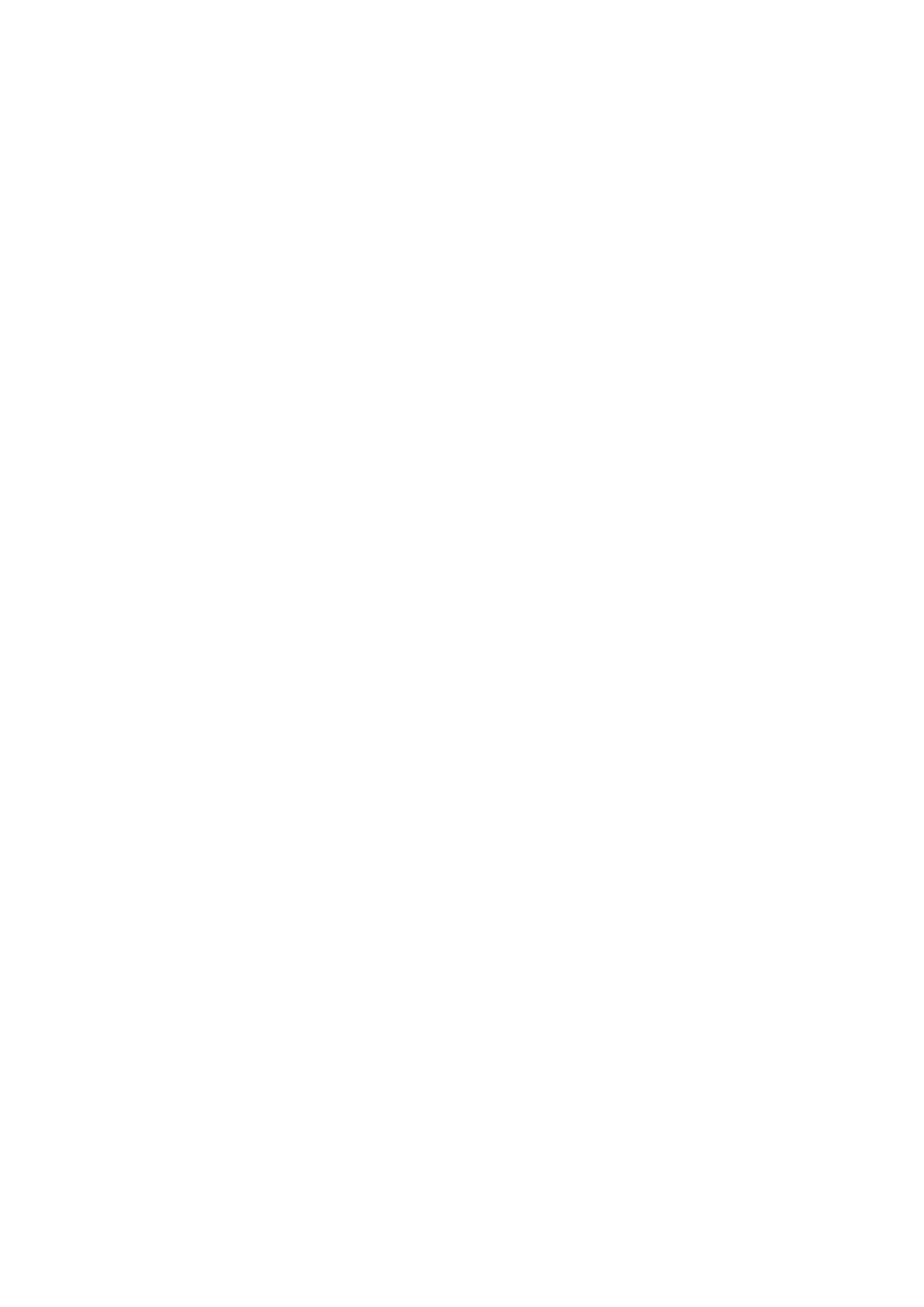
Table des matières
Liste des abréviations 1
IQuelques développements thématiques 7
1 Thèmes de recherche 9
2 Calcul et interconnexions optiques tridimensionnels 15
2.1 Degrés de liberté spatiaux d’un front d’onde . . . . . . . . . . . . . . . . . 16
2.2 Système d’interconnexions tridimensionnelles . . . . . . . . . . . . . . . . 18
2.2.1 Modèlegénéral............................ 18
2.2.2 Codage optique du tenseur d’interconnexions . . . . . . . . . . . . 20
2.3 Transformations géométriques holographiques . . . . . . . . . . . . . . . . 21
2.3.1 Contexte ............................... 21
2.3.2 Approche itérative . . . . . . . . . . . . . . . . . . . . . . . . . . 23
2.3.3 Généralisation : transformations non-analytiques . . . . . . . . . . 24
2.4 Réseau de calcul à plan de nœuds . . . . . . . . . . . . . . . . . . . . . . . 27
2.4.1 Principe................................ 27
2.4.2 Implantation optique du RIPN et résultats expérimentaux . . . . . . 30
2.5 Conclusions et perspectives . . . . . . . . . . . . . . . . . . . . . . . . . . 34
3 Interconnexions et aiguillage optique entre fibres monomodes 37
3.1 Contexte et état de l’art . . . . . . . . . . . . . . . . . . . . . . . . . . . . 38
3.1.1 Réseaux WDM et transparence optique . . . . . . . . . . . . . . . 38
3.2 Principe de l’aiguilleur optique . . . . . . . . . . . . . . . . . . . . . . . . 41
3.2.1 Cahierdescharges .......................... 41
3.2.2 Interconnexions 1×Ndefibreàfibre................ 42
3.3 Déflexion de faisceau rapide à cristal liquide ferroélectrique . . . . . . . . . 45
3.3.1 Déflexion de faisceau digitale . . . . . . . . . . . . . . . . . . . . 45
3.3.2 Déflexion 2D et niveau d’intégration . . . . . . . . . . . . . . . . . 49
3.3.3 Cristaux liquides et cellule SSFLC . . . . . . . . . . . . . . . . . . 50
3.3.4 Modulation spatiale et réseau de biréfringence diffractant . . . . . . 51
3.3.5 Adressage, bistabilité et transparence optique . . . . . . . . . . . . 53
3.4 Démonstrateurs d’aiguillage optique 1D : résultats . . . . . . . . . . . . . . 56
3.4.1 Phase 1 : modulateur FLC 1×256pixels............... 56
3.4.2 Phase 3 : aiguilleur optique 1×14fibres............... 57
1