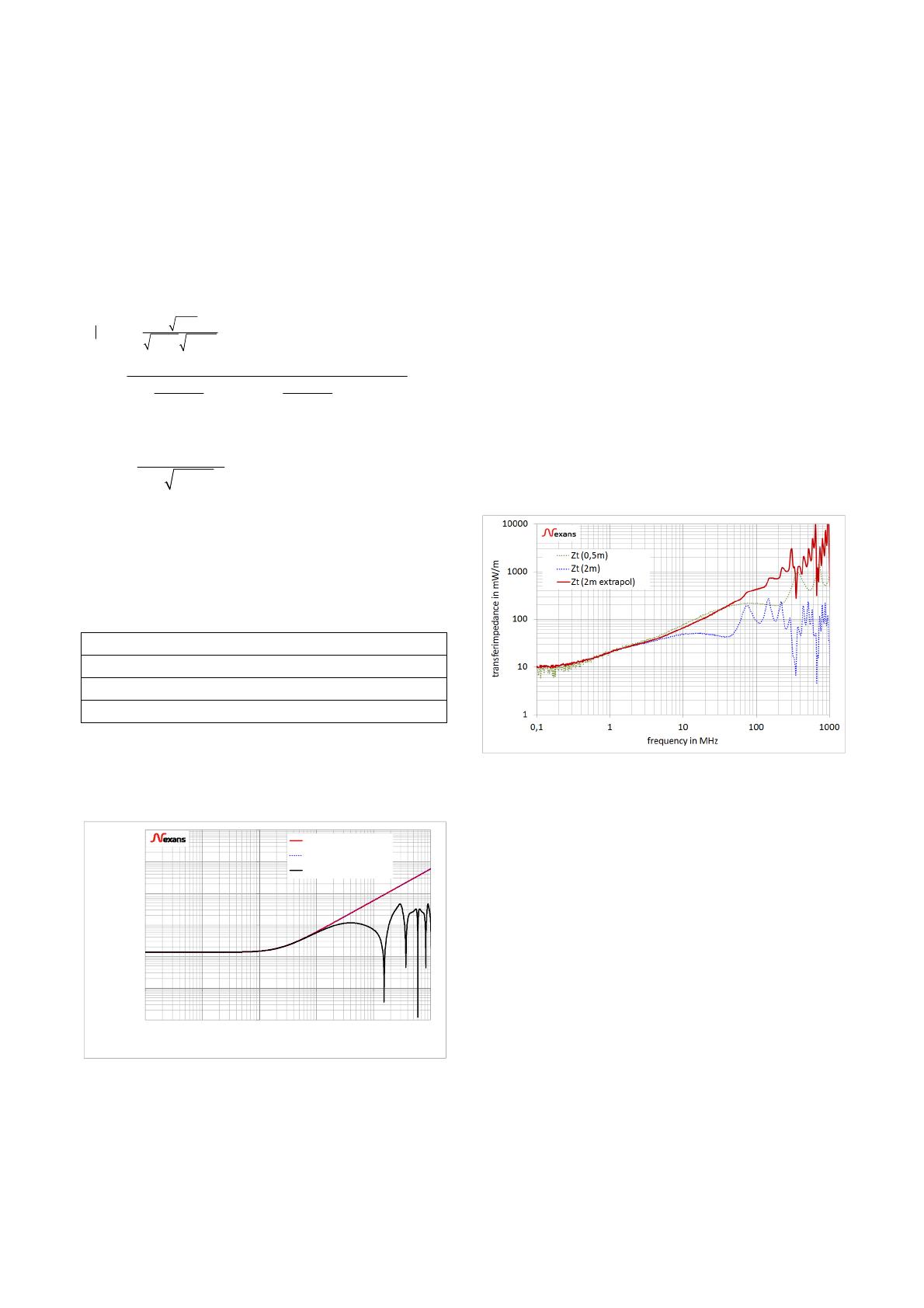
convert the measured scattering parameter SR41 to the transfer
impedance ZT.
3.2 Conversion between resulting (measured)
scattering parameter SR41 and transfer
impedance ZT
In most cases the capacitive coupling through the cable screen can
be neglected. This is in particular true for screens composed of
metal foils, dense single braids or double braids. In this case
formula (24) can be rearranged to obtain the transfer impedance ZT:
41
12
12
12 12
12 12
2
12
022
12
2
22
11 2 11 2
11
12 12
2
11
1
11
11
F
TR
Z
nf
L
LL
nf f nf f
LL
LL
L
ff
ZZ
ZS
rr
rre r e rrr e
ee
ere re
(25)
For low frequencies (L<<1) formula (25) can be rewritten as:
41
21 1
12
2
nf
TR
nf
ZZ
ZS LZZ
(26)
Figure 9 shows the comparison between the results of transfer
impedance obtained from formula (25) and the commonly used
conversion formula between the measured forward transfer
scattering parameter and transfer impedance as described in IEC
62153-4-3 [2]. The configuration of the triaxial setup is shown in
Table 3 with a mismatched inner circuit having a short circuit at the
far end.
Table 4: parameters for simulation of triaxial set-up
Z0=50Ω ; L=0,5m
Z1= 75Ω ; Z1,n= Z0 ; Z1,f= 0 ; εr1=2,25 ; α1=0
Z2=150Ω ; Z2,n=0Ω ; Z2,f=Z0 ; εr2=1,0 ; α2=0
RT=13,6mΩ/m ; MT=0,93nH/m ; CT=0
The transfer impedance obtained from formula (25) corresponds, as
expected, to the transfer impedance obtained from the screen
parameter (RT, MT, and CT). But using the formula described in IEC
62153-4-3 to convert the measured forward transfer scattering
parameter to transfer impedance limits the upper frequency for the
transfer impedance to about 30MHz.
Figure 9: comparison of formulae for conversion
between forward transfer scattering parameter and
transfer impedance
In formula (25) it is necessary to know the propagation constant of
the inner and outer circuit. A method to obtain them from
measurement is described in IEC 62153-4-16 [8].
4. Measurement versus simulation results
Figure 10 shows the results of the conversion from the measured
scattering parameter SR41 to the transfer impedance for a RG59 type
cable. The DUT was matched at the near end (generator side) and
far end. The conversion was done using a relative dielectric
permittivity of the inner circuit (DUT) of 2,3 (PE dielectric) and 1,1
of the outer circuit (PVC jacket in tube). The blue line and the green
line show the transfer impedance when measured with a 2m
coupling length respectively 0,5m and using the standard
conversion formula as described in [2] and formula (26). One can
see that the upper frequency limit for the validity of the transfer
impedance is 10MHz for the 2m length and 40MHz for the 0,5m
length. The red line shows the conversion to transfer impedance of
the 2m length using formula (25). Here the upper frequency limit
for the validity of the transfer impedance is 200MHz. The observed
peaks at higher frequencies are due to the capacitive coupling
impedance ZF which is not exactly zero (single braid with “low”
coverage) and due to uncertainties in the dielectric permittivity used
in the conversion formula (25).
Figure 10: Example for the conversion of measured
scattering parameter SR41 to the transfer impedance of a
RG59 type cable; inner circuit matched; assuming
relative dielectric permittivity of 2,3 and 1,1 for the
inner, respectively outer circuit
Figure 11 shows the test results of transfer impedance for a single
braided coaxial cable with 50Ω impedance. The DUT is short
circuited at the far end (Z1f=0). The results are shown for 3
different coupling lengths 35cm, 100cm and 200cm. The
conversion from the measured scattering parameter SR41 to the
transfer impedance was done according to IEC 62153-4-3 [2]. The
results show that the cut-off frequency for the transfer impedance
is decreasing with increasing length from 60MHz for 35cm to
10MHz for 200cm.
The IEC standard 62153-4-16 [8] to extend the frequency range is
only applicable for a matched DUT and cannot be used for a DUT
with a short circuit at the far end. The newly introduced equation
(25) however can be used for any load conditions. This is shown
for the above cable in Figure 12. The results for the coupling
length of 100cm and 200cm have been extrapolated using
equation (25). The conversion was done using a relative dielectric
permittivity of the inner circuit (DUT) of 2,3 (PE dielectric) and
1,0 of the outer circuit (cable jacket was removed). The cut-off
0
1
10
100
1000
10000
100000
0,01 0,1 1 10 100 1000
transferimpedanceinmilli‐Ohm/m
frequencyinMHz
ZTofscreen
ZTfromS41
ZTfromIEC62153‐4‐3