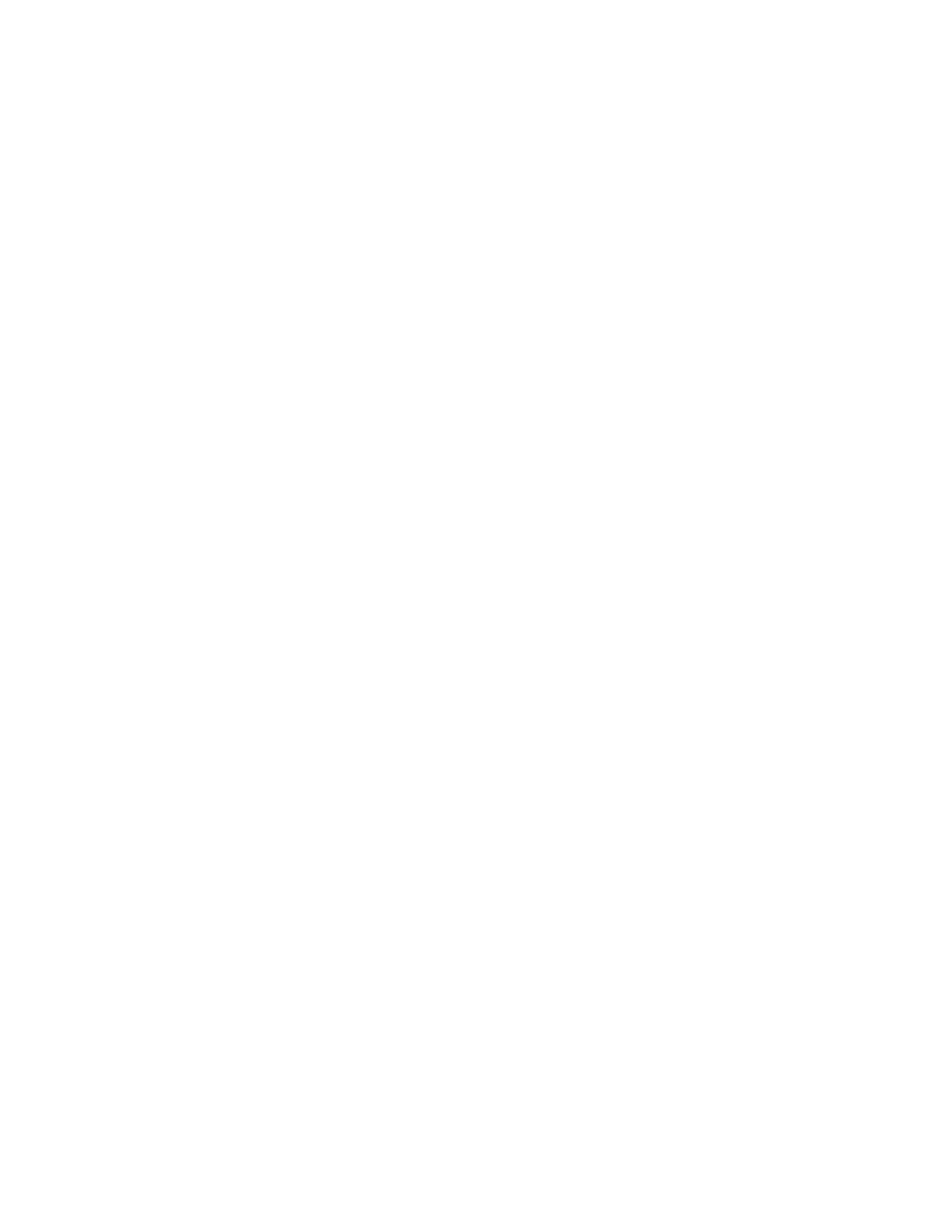
v
RÉSUMÉ
Dans cette thèse de doctorat, les réseaux de nano-trous périodiques dans des films métal-
liques sont étudiés. Leurs dimensions sont de l’ordre de la centaine de nanomètres, autant
pour la taille du trou que l’espacement entre chacun et l’épaisseur du film. Ils possèdent
des propriétés optiques remarquables : à certaines longueurs d’onde, la lumière qui diffracte
à la surface de cette structure peut se coupler avec l’onde de propagation à l’intérieur du
trou. Lorsque ces conditions sont rencontrées, un phénomène de résonance est excité et une
forte transmission optique est observée de l’autre côté du film. On parle alors de transmis-
sion optique extraordinaire ou EOT pour "Extraordinary Optical Transmission". Le terme
extraordinaire provient du fait que, si on considère la transmission optique des trous indivi-
duellement, l’intensité observée serait plusieurs ordres de grandeur inférieure à celle mesurée
pour le réseau. De plus, même si on réunissait tout les trous en un seul grand trou (avec
les proportions avec la partie métallique conservée), la transmission optique serait encore
inférieure à celle observée pour le réseau. Le réseau possède donc la propriété de canaliser la
lumière vers les indentations et ainsi de concentrer l’onde électromagnétique vers l’intérieur
de l’indentation. Ces structures permettent de manipuler la lumière à l’échelle du nanomètre
et ainsi de construire des matériaux avec des propriétés optiques remarquables.
Dans une première partie de cette étude de ces structures, la modélisation des propriétés
optiques est abordée en utilisant la théorie des modes couplés ou CMT pour "Coupled Mode
Theory". Cette méthode consiste en une expansion des champs électromagnétiques des modes
possibles dans chacun des milieux de la structure ainsi qu’un calcul des coefficients de cou-
plages de l’onde d’un milieu à l’autre. Bien que d’autres méthodes de modélisation analytique
existent, la CMT a été choisie car elle permet de minimiser les approximations employées
dans la modélisation, tout en offrant un développement analytique typique des problèmes de
transmission optique. De plus, sous certaines approximations, la méthode permet d’écrire les
équations sous une forme simple qui décrit directement l’origine de la résonance. Une com-
préhension approfondie des mécanismes en jeu de la transmission optique est donc obtenue
grâce à cette modélisation.
Dans le cadre de ce travail de modélisation, une contribution originale a été effectuée afin
d’approfondir la méthode lorsque l’on considère plusieurs modes possibles à l’intérieur des
indentations. Effectivement, uniquement des approches approximatives sont disponibles dans
la littérature, ou des approches utilisant uniquement le mode fondamental guidé à l’intérieur
du trou, qui sont des approximations valides uniquement dans le cas sous-longueur d’onde ou