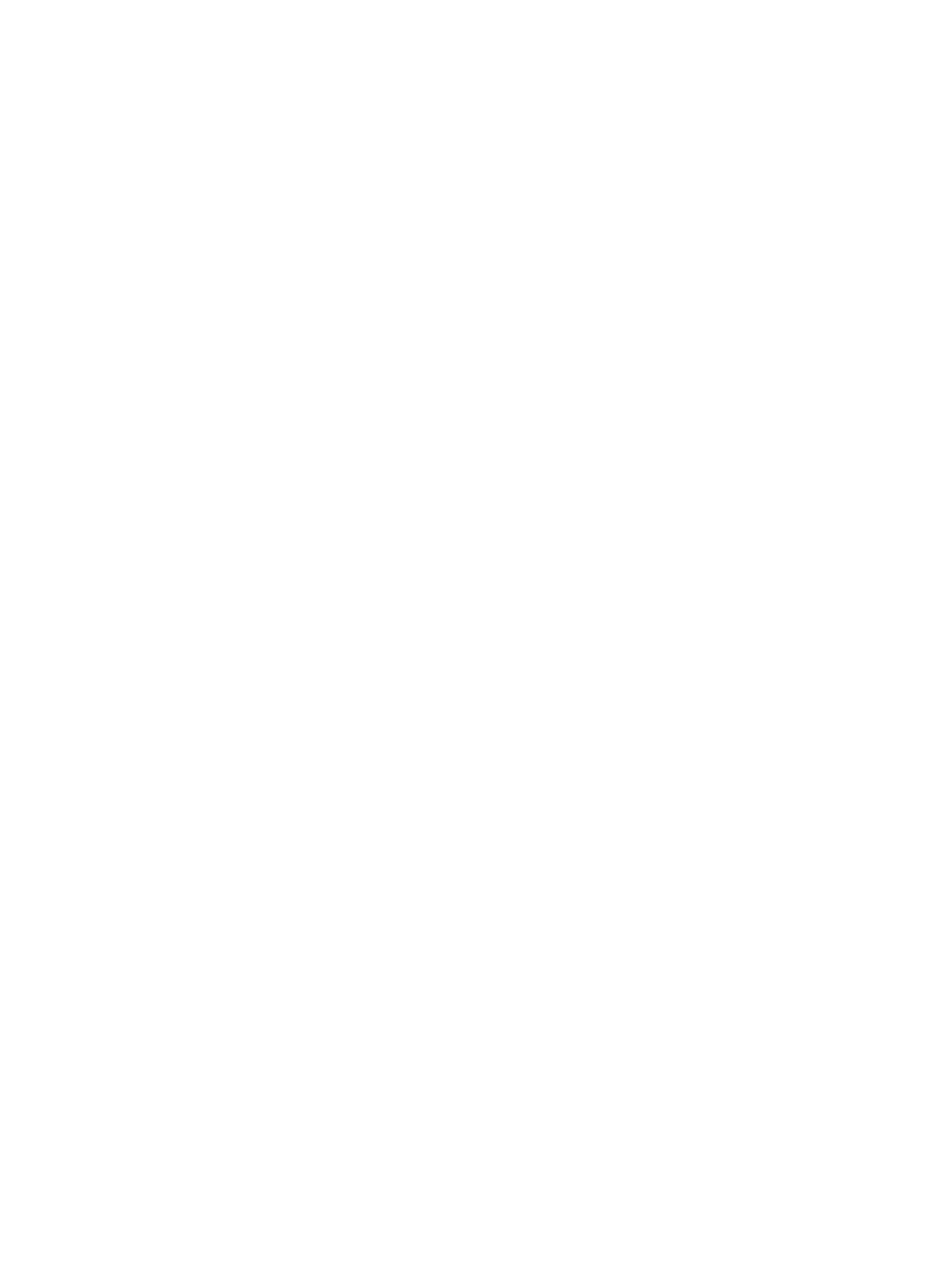
2. Material and methods
2.1. Sample preparation and drying
Experiments were performed on the potato (Eureka) samples
collected from a local market in Brisbane, Australia. Samples were
stored in a refrigerator at 4
C until the start of drying experiments,
which were performed in a cabinet dryer. The dryer was started
about 30 min before drying experiments to achieve steady state
conditions before each drying run. At the start of each experiment,
the materials were washed and cut into cylindrical slices of 30 mm
thickness and 20 mm diameter. Experiments were performed at a
temperature of 60
C and a constant air velocity of 0.7 m/s. The
relative humidity of the inlet air was in the range of 60%e65%.
During the drying process, the tray was taken out at 30-min in-
tervals and weighted using a digital electronic balance (model
BB3000; Mettler-Toledo AG, Grefensee, Switzerland). The mea-
surement range of the balance was 0e100 g with an accuracy of
0.01 g. The temperature was measured by a thermal imaging
camera Flir-i7. The temperature range of the thermal camera
was 20
C to 250
C with 140 140 pixels resolution. In order to
better interpret and analyse the results, a simulated 3D tempera-
ture distribution profile was developed (Fig. 7) using a validated
heat transfer model. The details of the theoretical model, from
where simulation results were generated, can be found in authors'
previous publications [25,26].
2.2. NMR measurements
At different stages of the drying process, the samples were taken
out of the dryer and immediately placed in a 25 mm diameter NMR
tube. To protect the sample from oxidation, it was immersed in
Fomblin PFPE (Grade 06/6, USA) oil to provide a barrier for mass
transfer between samples and surroundings and to assist with
sample shimming. The tube was sealed with a standard NMR tube
cap and incubated at 22
C for 5 min to reach thermal equilibrium.
Measurements were made with a Bruker DRX wide-bore spec-
trometer (Bruker Bio-spin, Karlsruhe, Germany) operating at
300 MHz for hydrogen and fitted with a micro-imaging (micro 120)
gradient set and a birdcage coil. Data were collected and processed
using Paravision 4 software (Bruker). Regional T
2
relaxation times
were measured from relaxation maps acquired with a multi-slice-
multi-echo (MSME) sequence using the following acquisition pa-
rameters: 64 averages, 1000 echoes with 10 ms echo time and 5.0 s
repetition time. The slice thickness and the matrix size were 3 mm
and 6464, respectively. The spatial resolution of the scans was
468
m
m.
2.3. Mathematical analysis
2.3.1. Theory
The water mobility in potato tissue was investigated quantita-
tively using multicomponent analysis of T
2
relaxation decay curves.
A nonlinear least-squares method was applied for data analysis
[27]. Generally, the free induction decay of the proton relaxation
follows an exponential decay. For multiple environments, there will
be corresponding T
2
relaxation time constants. A multi-exponential
equation can describe these functions. Each tissue compartment
corresponds to a different environment and will have a distinct
relaxation time constant (T
2
). It was assumed that these compart-
ments were not inter-reliant at the time of the measurements such
that the multi-exponential nature of the T
2
decay curve relates to
the different water compartments in the tissue, and the water
molecules do not undergo rapid exchange between compartments
on the NMR timescale. However, when water proton relaxation
follows a pattern of mono-exponential decay, there is a fast ex-
change of protons between tissue water and macromolecules,
showing that the water compartments are symbiotic [28e30].
2.3.2. Data analysis
For each sample, two regions-of-interest (ROI) were defined
manually on a given slice of the mid transverse section of the MSME
images [6]. The mean value of these ROIs was computed for each
sample. The accuracy of the multi-exponential parameters
considerably depends on the noise level; therefore, a third ROI was
assessed outside the sample for determining the signal-to-noise
ratio (SNR, average signal intensity over the standard deviation of
the noise). Noisy T
2
-signals were eliminated from the original
signal, as described in authors' previous publications [6,31], and
data with appropriate SNR (SNR>5) were used for parameter
fitting. In order to achieve SNR >5, a constant cut-off of TE <600 ms
was used for all datasets [32]. The mean signal of the two ROIs at
each echo time were measured and plotted as a function of time on
a semi-log scale where curvature was determined [33]. The number
of inflection points was used to determine the number of T
2
com-
ponents. The different T
2
relaxation times comprising the signal
from each sample was determined by bi-exponentially fitting the
mean signal intensity using the following equation.
Y¼A1expt=T
1
2
þA2expt=T
2
2
(1)
where, Yis the function of T
2
relaxation time constant, A
1
and A
2
represent the relative contributions of the two proton environ-
ments, and T
1
2
,T
2
2
are the relaxation time constants of the different
components. For T
2
measurements, odd echoes were excluded to
minimize error due to the influence of stimulated echoes [6,34,35].
T
2
relaxation time data processing was carried out with a non-
negative least squares algorithm using self-written program code
in the curve fitting toolbox of Matlab
®
software (The MathWorks,
Inc., Natick, MA). The experiments were replicated three times for
each sample, and the average of these measurements was used for
analysis. The details of the experimental procedure can be found in
the authors' previous publication [6].
2.4. Statistical analysis
Data are expressed as mean ±SD of the mean. For statistical
analysis least-squares linear regression analysis was used. A 95%
confidence level was regarded as significant, as shown in Table 1.
3. Result and discussions
The water migration mechanisms at different stages of drying
were investigated using
1
H-NMR relaxometry. The T
2
relaxation
decay curves obtained from samples at different drying times are
presented in Fig. 3. Each curve was fitted using the multi-
exponential decay equation (1). After fitting the bi-exponential
decay curve with different T
2
relaxation intensity data, two
different proton components of water relaxation (long and short)
were determined, as shown in Table 1, with their corresponding
standard deviation and statistical confidence level. Depending on
the water mobility and pore size, two signal components namely,
long and short were categorised as intracellular environmental
water (BW) and intercellular environmental water (FW) [6].
3.1. Collapse of the cell membrane during drying
Fig. 4 shows the change in the proportion of BW over time
during the drying process, calculated on the assumption that the
total moisture present at that time is considered 100%. The curve is
M.I.H. Khan et al. / International Journal of Thermal Sciences 117 (2017) 266e273268