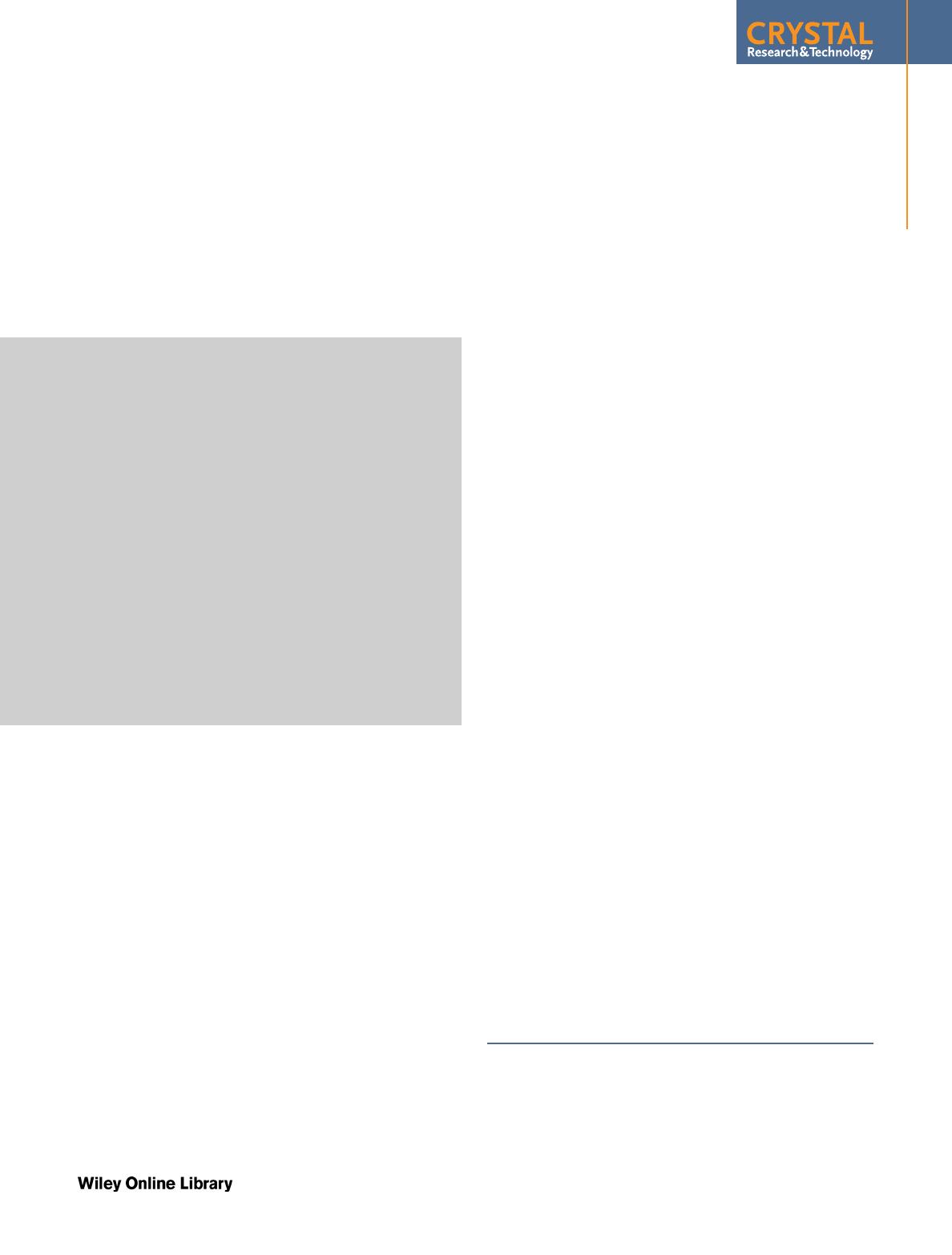
Cryst. Res. Technol. 49, No. 11, 899–906 (2014) / DOI 10.1002/crat.201400231
Original Paper
Fabrication of hollow ZnO nanostructures by a CTAB-assisted
chemical bath deposition method
Ying Hou and Ming Yang∗
Received 8 July 2014, revised 4 September 2014, accepted 15 September 2014
Published online 9 October 2014
ZnO lms consisted of hollow nanostructures were prepared
by a CTAB-assisted chemical bath deposition (CBD) method.
ZnO rings, bowls and assemblies of hollow structures were
successfully obtained on dierent substrates. Dense ZnO
lms consisted of sunken prisms can also be achieved by
controlling the concentration of CTAB. The inuences of
reactant concentrations, types of the substrates and pre-
coated ZnO nanoparticles on the formation of ZnO lms
were examined. XRD patterns indicated the Wurtzite struc-
ture of ZnO and the preferred growth direction is [001]. The
role of CTAB in CBD process was discussed and the evolution
of dierent ZnO nanostructures was studied based on the
observation of SEM. A plausible crystal growth mechanism
was proposed for the formation of ZnO rings and bowls. The
investigation of optical properties showed that high con-
centration of CTAB can improve the ultraviolet emission.
1 Introduction
The shape, size and crystalline structure of semi-
conductors are important elements in determining
their chemical and physical properties [1]; to meet
the actual technological applications, the rational
control over these factors are essential in today’s ma-
terial science [2]. The wide direct band-gap of ZnO
(3.37 eV) and large exciton binding energy (ca. 60 meV
at room temperature) makes it a promising optoelec-
tronic material in the UV region [3]. Other important
properties including size-dependent surface lumines-
cence [4], room-temperature storage of hydrogen [5],
photocatalysis [6], nanoelectricity generators [7] have
also been demonstrated for ZnO-based materials.
Accordingly, various ZnO nanostructures have been
synthesized including nanowires [8], nanotubes [9],
nanosprings [10], nanonails [11], tetrapods [12], and
etc. using high-temperature physical methods. The
solution-based technique provides an environment
friendly and economical way in controlling the mor-
phologies of ZnO nanostructures. Several interesting
structures have been obtained via solution methods,
such as hexagonal discs and rings [13], doughnut-
shaped microparitlces [14], nanowalled microboxes [15],
helical rod-like structures [16], nanopyramids [17],
nanosheets [18], and hierarchical structures [19]. The
chemical bath deposition (CBD) of metal oxide thin
films, which is based on controlled precipitation on a
substrate via hydrolysis and/or condensation reactions
of metal ions and/or complexes from aqueous solu-
tion [20], is effective for the preparation of orientated
ZnO nanorod and nanotube arrays which have impor-
tant applications in nanoscale devices [21]. However,
there are very limited investigations on the influence
of surfactants during the CBD process, which may play
an important role in the formation of ZnO nanostruc-
tures. Further, hollow nanostructures can have special
advantages when compared with solid structures due
to their higher specific surface area [22]. In this study,
a cetyltrimethylammonium bromide (CTAB)-assisted
CBD process was used to controllably prepare ZnO with
different morphologies, such as ZnO rings and bowls.
ZnO films consisted of dense sunken prisms can also be
obtained. Based on the study of the influence of different
reaction conditions, the role of CTAB in CBD process was
discussed and a possible formation mechanism of these
ZnO structures was proposed. The photoluminescence
(PL) spectra of ZnO samples were also investigated.
Key Laboratory of Microsystems and Micronanostructures Manu-
facturing, Harbin Institute of Technology, 2 Yikuang Street, Harbin,
150080, P. R. China
899
C2014 WILEY-VCH Verlag GmbH & Co. KGaA, Weinheim