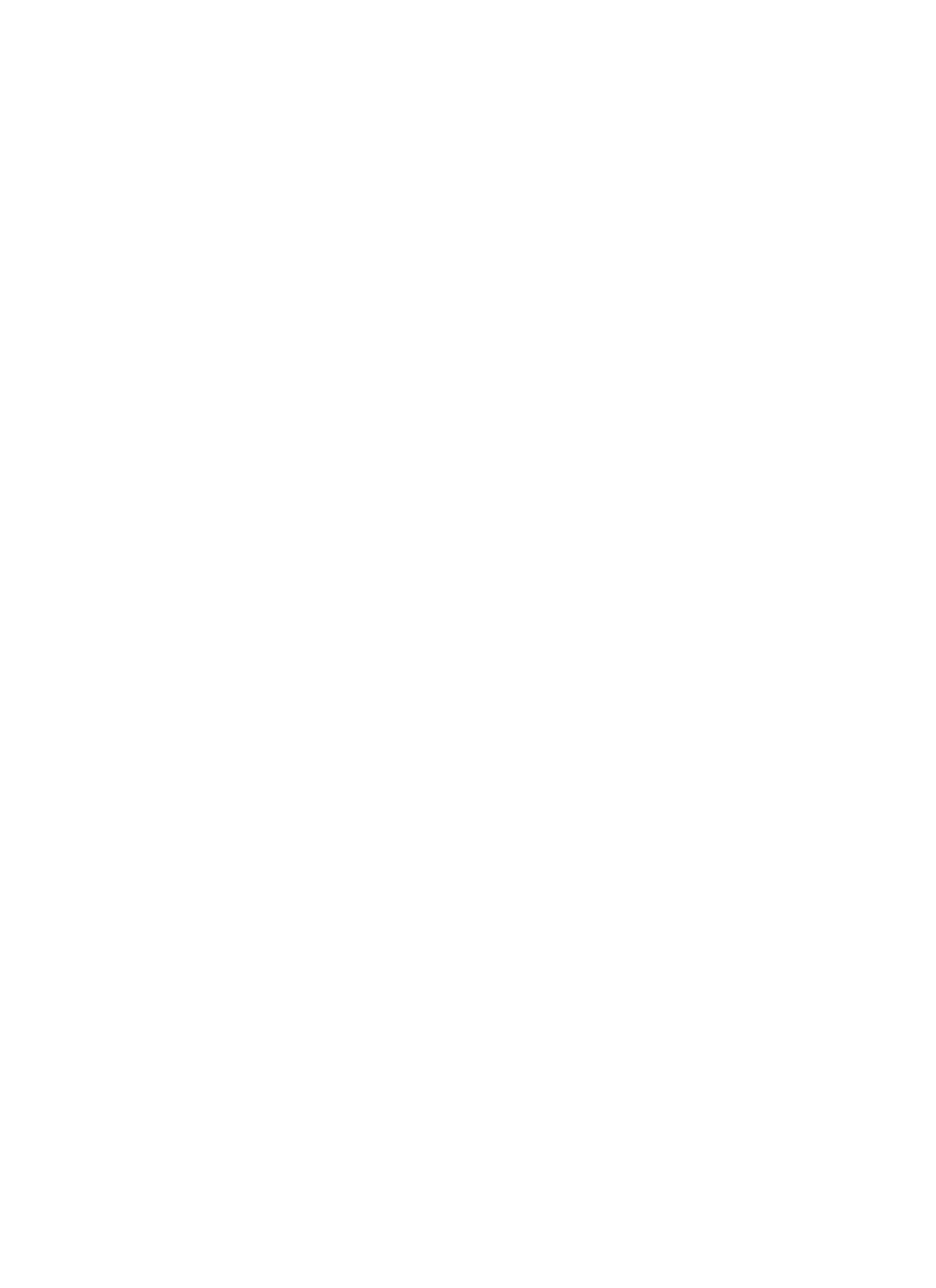
The size of upland aquifers controls the magnitude
and seasonality of subsurface flow and elements
inputs to riparian areas (Hill, 2000). The threshold
between riparian zones that are seasonally and
permanently connected to uplands may be the result
of small differences in average depth of upland
storage in some landscapes (Devito et al., 1996).
The size and seasonality of upland hydrologic
connection also influences riparian zone water table
fluctuations and the extent of surface saturation with
consequent effects on soil microbial redox reactions
and water chemistry (Roulet, 1990; Hill and Devito,
1996; Devito and Hill, 1997).
The depth and permeability of saturated sediments
overlying a confining layer in riparian zones can
influence riparian hydrologic flowpaths, water
residence times and renewal rates (Schnabel et al.,
1994; Correll et al., 1997). A confining layer at a
shallow depth increases the interaction of subsurface
flow with vegetation root systems and surface soils
and enhances the potential of the riparian zone for
nitrate removal (Hill, 1996). In riparian zones where a
confining layer is absent, groundwater flow at depth
may bypass the riparian zone and discharge directly to
the stream channel (Bohlke and Denver, 1995).
Gravel layers in the soil profile beneath less
permeable sediments can be zones of preferential
subsurface flow across the riparian zone (Burt et al.,
1999). Conversely, sediments with low hydraulic
conductivities (e.g. peats, clays in oxbow channels)
can divert water upward or downward altering
subsurface flowpaths in the riparian zone (Hill,
1990; Brusch and Nilsson, 1993; Burt, 1997).
Research also indicates that topography affects the
hydrological functioning of riparian zones (Devito
et al., 2000a). The slope gradient, especially at the
riparian zone-upland margin, influences the hydraulic
gradient and the volume and velocity of water
entering the riparian zone. A concave riparian profile
typically favours interaction between subsurface
water and superficial soil horizons where denitrifica-
tion and root uptake are likely to occur, whereas
convex topography favours deeper water tables and
subsurface flowpaths. Burt et al. (2002) have shown
that when the riparian zone is flat, the stream water
level acts as a fixed point around which the water table
fluctuates, whereas when the slope directly borders
the river, the effect of stream water level on water
table dynamics in the riparian zone is negligible.
Although the importance of landscape hydrogeo-
logic setting is recognized, researchers have not
studied whether characteristics such as upland aquifer
thickness, topography and riparian lithology can be
used together to understand and predict the
hydrological functioning of riparian zones. In order
to develop a better understanding of how these
landscape characteristics control riparian zone hydrol-
ogy, we need to conduct a comparative analysis of
riparian zones in landscapes that differ in hydro-
geologic characteristics. With the exception of a
recent study of water table fluctuations in European
riparian zones by Burt et al. (2002), researchers have
examined single riparian sites.
In this study, we examine the effect of upland
aquifer size, topography and riparian sediment
lithology on the subsurface hydrology of eight
riparian sites located on glacial till and outwash
landscapes in southern Ontario, Canada. The riparian
zones were selected to provide a range of sediment
permeability, slope gradients and upland aquifer
depths. The comparison of the hydrology of
these riparian zones focuses on two main questions:
(1) what is the influence of increasing upland
aquifer size on the magnitude and duration of
upland–riparian hydrologic linkages? (2) how do
hillslope discharge, topography and riparian sediment
lithology interact to influence water table fluctuations,
riparian groundwater flow patterns and fluxes entering
the riparian zones? Our understanding of riparian
hydrology is used in the concluding section of the
paper to develop a conceptual framework of different
classes of riparian groundwater dynamics based on
hydrogeologic setting to generalize about riparian
zone hydrology at the landscape scale.
2. Study sites
The study sites are located in agricultural
catchments in southern Ontario near Toronto
(Fig. 1). The annual precipitation in the area is
800–900 mm/yr with 120 –240 mm falling as snow
between December and April (Singer et al., 1997).
The mean annual temperature is 7.2 8C with a mean
January temperature of 26.7 8C and a mean July
P.G.F. Vidon, A.R. Hill / Journal of Hydrology 292 (2004) 210–228 211