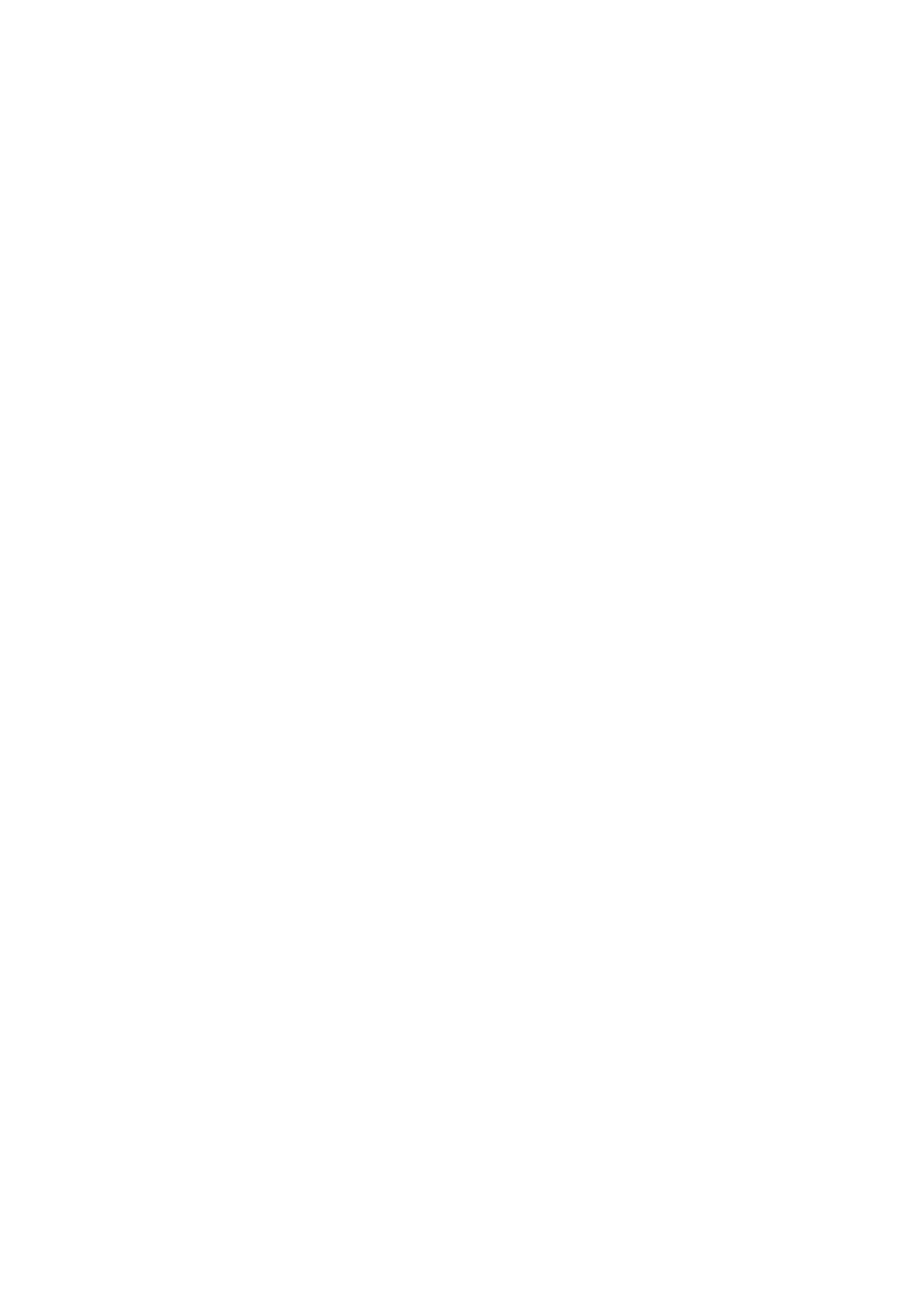
2
AGRADECIMENTOS
À Profa. Dra. Ana Luiza Maia, a grande responsável por este trabalho, os meus
agradecimentos pela orientação, pelas inúmeras oportunidades, por toda a confiança,
paciência e amizade. Obrigada por ter apostado em mim e contribuído de maneira
extraordinária para a formação da pessoa que sou hoje.
Aos colegas e muito mais do que isso, amigos do Grupo de Tireoide, Lucieli Ceolin,
Carla Vaz Ferreira, Simone Wajner, Rafaela Vanin Pinto Ribeiro, Helena Cecin Rohenkohl,
Carla Krause, Shana Weber, Juliano Dalla Costa, Clarissa Capp, Leonardo Leiria, Debora
Rodrigues Siqueira, Nadja Zennig, Rafael Selbach Scheffel e José Miguel Dora pela preciosa
amizade e por compartilharem tantos momentos agradáveis no laboratório, vocês
simplesmente tornam meus dias mais coloridos. Obrigadas especialmente àqueles que
contribuíram ativamente nas diferentes etapas deste trabalho.
Aos colegas do laboratório de biologia molecular do Serviço de Endocrinologia pelo
excelente ambiente de trabalho e pela cooperação em diversos momentos da execução desta
tese.
À querida amiga Patrícia Lopes pela paciência e pelo enorme auxílio teórico e prático
nos experimentos de citometria de fluxo.
À Profa. Dra Edna Teruko Kimura e ao Dr. César Seigi Fuziwara por terem
proporcionado um período de intenso aprendizado, pela paciência e pela efetiva contribuição
neste trabalho.
À minha amiga/irmã Alessandra Kherkoff pela amizade inestimável, pelas palavras
certas em todos os momentos e pelo apoio incondicional.
Aos meus pais, à minha irmã Francielle, ao meu sobrinho Lorenzo por todo o amor,
pelo carinho e pela força. Obrigada por vocês serem o alicerce da minha vida.
Ao meu companheiro Anderson Milani pelo amor, paciência, compreensão e apoio
incondicional.