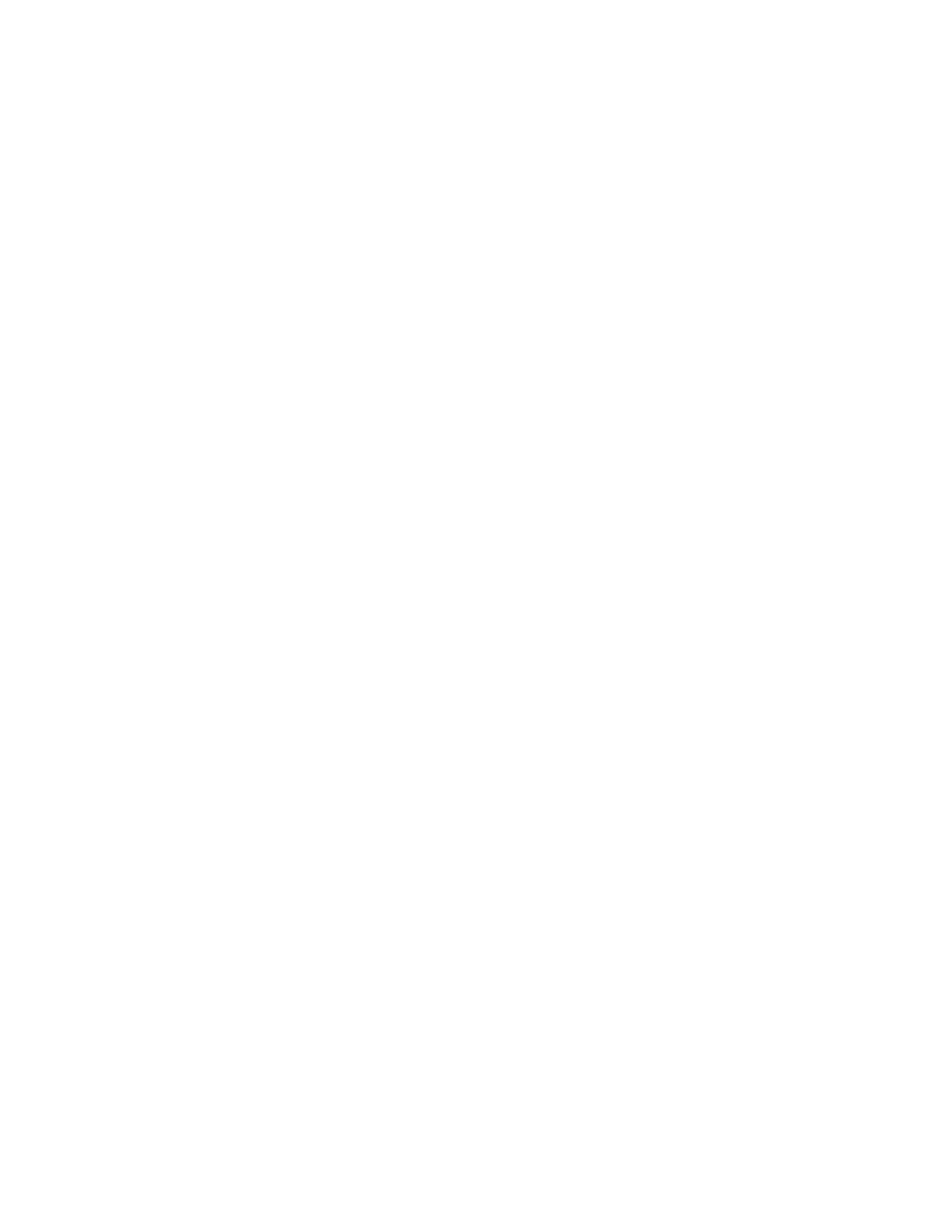
4
in euthyroid animals or in cell cultures 3,5-T2is less potent than T3(see Goglia 2005 for
review).
One prevailing question is how 3,5-T2is formed in the target cell. Although
conversion of T3to 3,5-T2has not been demonstrated in mammals (Maia et al. 2005) or
in teleosts in vitro (A. Orozco, unpublished observations; Sweeting and Eales 1992),
indirect but robust evidence indicates that 3,5-T2is indeed formed from T3in vivo
through deiodination (Moreno et al. 2002). It is not yet known which deiodinase
catalyzes the conversion of T3to 3,5-T2either in vivo or in vitro,but a likely candidate is
iodothyronine deiodinase type 2 (D2). D2 catalyzes the removal of outer-ring iodine from
thyroxine (T4)to generate the bioactive hormone T3and thus determines T3availability
at the cellular level. Conversion of T3to 3,5-T2would require similar outer-ring
deiodination.
We have previously studied whether 3,5-T2has a regulatory effect upon D2 in
Fundulus heteroclitus (killifish). In this species, hepatic D2 expression is very abundant,
amongst the highest in vertebrates so far examined (Orozco et al. 2000; Orozco and
Valverde-R 2005). The D2 gene is tightly down-regulated by bioactive TH (for review:
Bianco et al. 2002; Bianco and Larsen 2005). We have found that hyperthyroidism
induced by a short-term immersion (up to 24 h) of killifish in seawater supplemented with
3,5-T2significantly decreased both mRNA expression and hepatic activity of D2,
mimicking the effects of T4or T3(Garcia-G et al. 2004). Because these effects were
obtained with supra-physiological doses of the three iodothyronines, in this work, we
asked whether 3,5-T2,when administered in doses similar to those known to be
physiological for T3,could re-establish the euthyroidal activity and hepatic expression of
D2 in hypothyroid killifish. We also examined the effects of 3,5-T2upon the hepatic
expression of growth hormone (GH), which is up-regulated in the pituitary by
Page 4 of 28