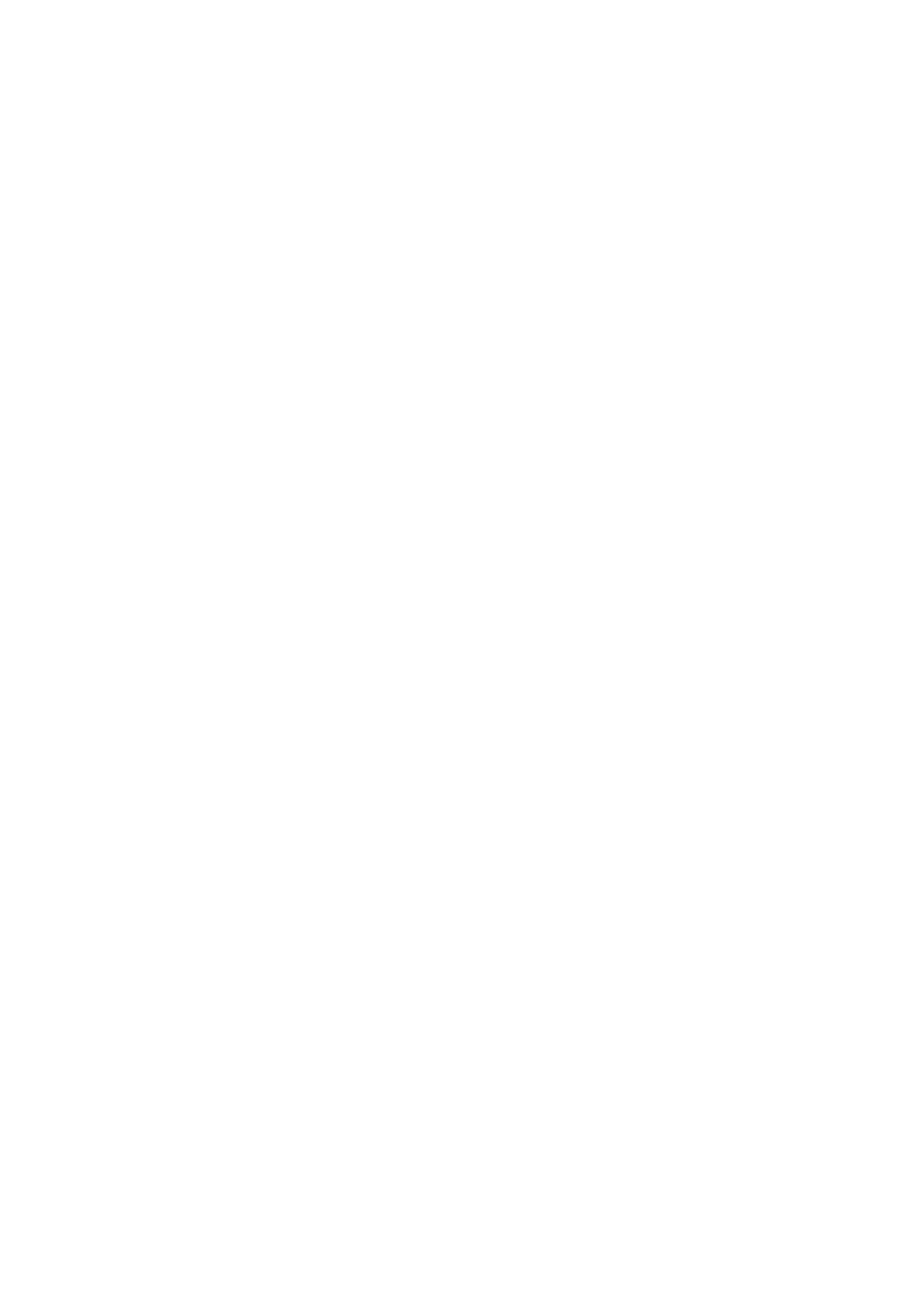
IV
AGRADECIMENTOS
Ao meu querido orientador, Prof. João Antonio Pegas Henriques, pela
confiança depositada em meu trabalho. Obrigada por acreditar em mim, por me
proporcionar imensas oportunidades e por contribuir com a ciência. Obrigada por
continuar acreditando e confiando em mim!
Ao meu co-orientador Alexandre Escargueil e à Prof. Annette K. Larsen, pelos
ensinamentos, discussões, ideias, oportunidades, convivência e paciência durante
o meu período de estágio-sanduíche na França.
À Danièlle Grazziotin Soares pela amizade e por todo o tipo de ajuda que eu
poderia e não poderia esperar durante minha estadia na Fraça.
Ao Prof. Guido Lenz que contribuiu mais do que significativamente para este
trabalho e sempre me incentivou, me inspirou e me ensinou muito sobre
autofagia.
Ao CNPq, à CAPES e à FAPERGS, pelo suporte financeiro que proporcionou a
realização deste trabalho.
Ao Programa de Pós-Graduação em Biologia Celular e Molecular pelas
oportunidades.
A todos os meus colegas que passaram pelo Laboratório 210 da Biofísica
durante esses anos: Cristiano, Ana Lúcia, Valéria, Larissa, Nusha, Albanin,
Fernanda, Clara, Brunas, Jaque, Dinara, Angelo, André, Renata, Fabrício, Iuri,
Grethel, Lyda, Bic!
Um agradecimento especial à Michelle de Souza Lima que foi bolsista de
iniciação científica do Laboratório 210 e trabalhou intensamente durante a
execução desta tese. Sem a ajuda da Michelle nada teria acontecido!
À minha amiga e colega Miriana Machado que sempre me apoiou de todas as
formas, me incentivou e teve muita paciência e compreensão.