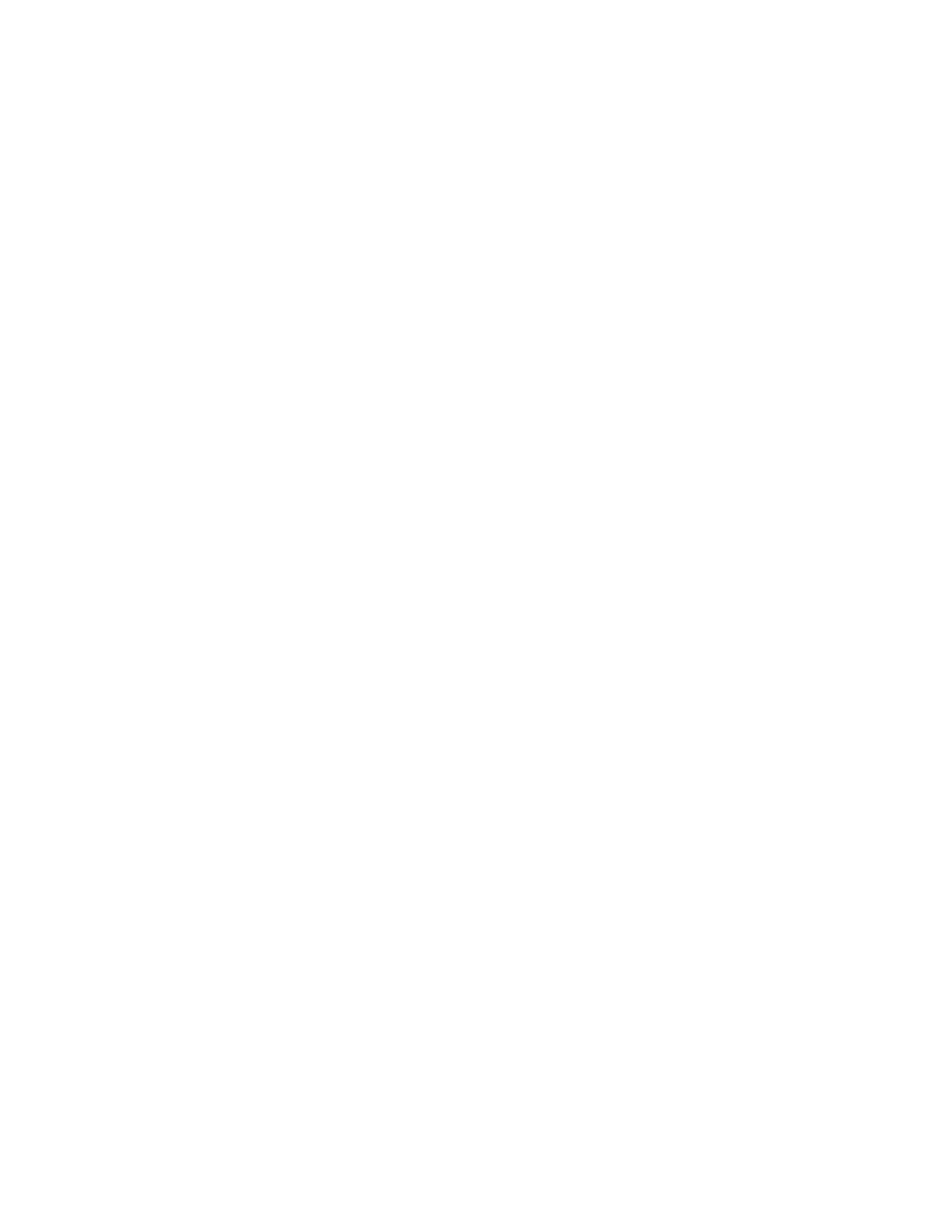
Acknowledgements
I would like to acknowledge the love and support of my family that has made it
possible for me to achieve everything that I have. My wonderful parents, Daljit
and Baljinder, have always nurtured and supported me to the best of their ability
and beyond. My brother and my best friend, Gharandip, has been a constant
companion, source of guidance and inspires me to life in service. The love of my
life, Navkiran, who went from being my girlfriend to fiancé and finally, wife, during
the course of my graduate study years, is my reason for being.
I would also like to acknowledge the support of my supervisor, Dr. Donna-Marie
McCafferty. Donna-Marie has provided me with invaluable mentorship and
guidance over the last 6 years. I would like to thank my supervisory committee:
Dr. Joe Davison, Dr. Paul Beck and Dr. Oliver Bathe. Under the combined
supervision of Donna-Marie and my committee, I have expanded my knowledge
of the scientific method and how to put it into practice. It has been a privilege to
be mentored and guided along in my career by these and many other individuals
I would like to thank all members of the McCafferty lab over the years, especially,
Ronald Chan, Dr. Rui Zhang, Dr. Ying Gao, Kelvin Ng and Dr. Maitham Khajah. I
thank Dr. Stephan Urbanski for his great help with my project.