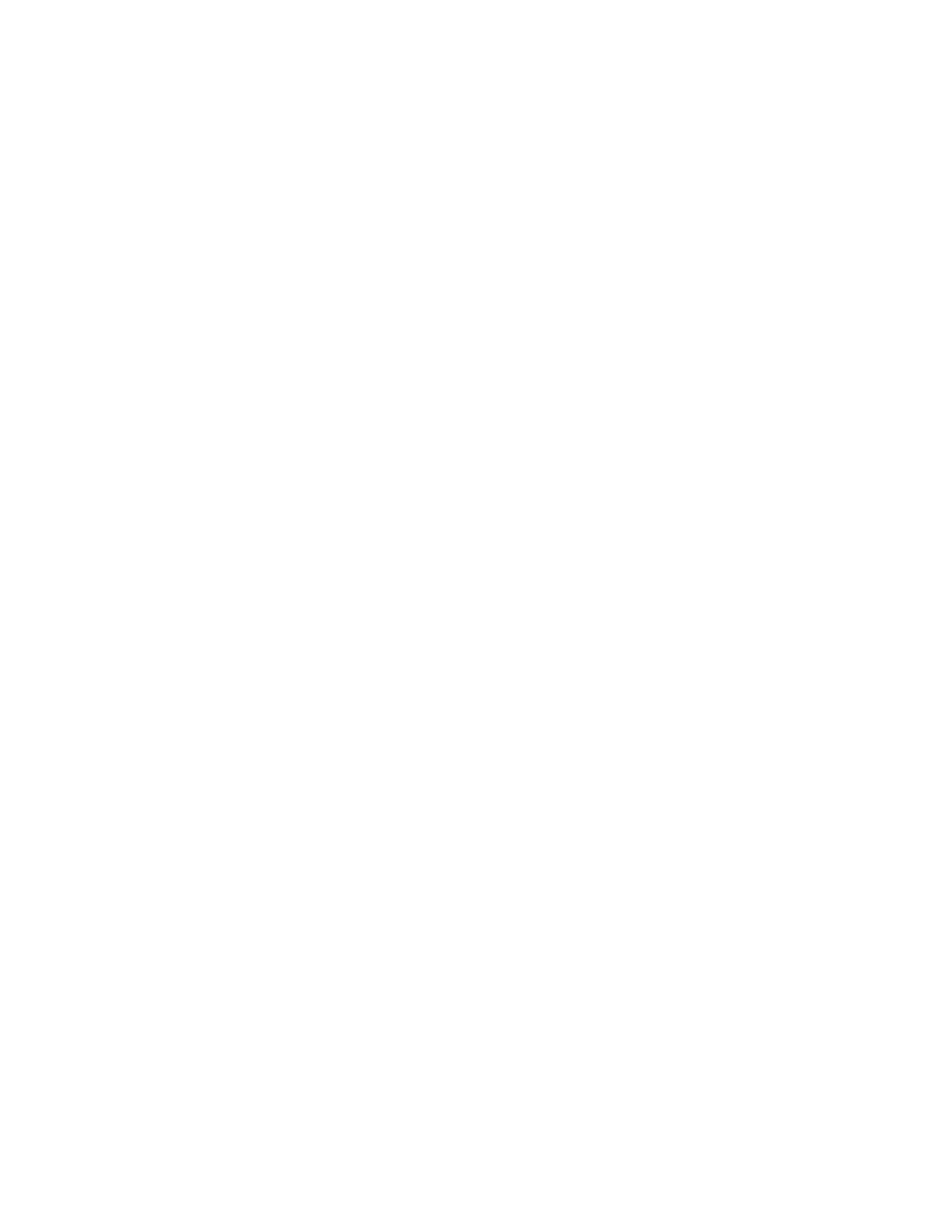
Leticia De Mattos-Arruda PhD thesis
Chapter 1: Introduction
Cell-free tumour DNA
In medical oncology, the next generation or massively parallel sequencing of tumour
tissue biopsies in search of actionable somatic genomic alterations has become routine
practice. Although the information derived from tumour tissue biopsies can be informative, the
procurement of tumour tissue specimens poses challenges for the development of biomarkers.
Tumour biopsies are single portraits of the tumour in time, can be difficult to obtain, can be
costly and time consuming, and subject to selection bias resulting from tumour heterogeneity (1,
2).
Blood-based circulating biomarkers, including circulating tumour cells (CTCs), cell-free
nucleic acids and exosomes, have been studied as ‘liquid biopsies’, that is, surrogates or
complementary biomarkers to overcome the drawbacks of invasive tissue biopsies (3). Recent
developments in massively parallel sequencing and digital genomic techniques have allowed for
interrogation of tumour-specific molecular alterations in the circulation and support the clinical
validity of cell-free circulating tumour DNA (ctDNA) as a liquid biopsy in human cancer (4-17).
Plasma is known to carry small amounts of fragmented cell-free DNA of 160 to 180 base
pairs, which is likely to be originated from cancer cells through the process of necrosis and
apoptosis (18-20). Tumour-derived DNA is defined by the presence of genomic alterations, and
can be discerned from normal DNA, reassuring the specificity of these liquid biopsies as
biomarkers in cancer (20). CtDNA in plasma constitutes a non-invasive source of material that
may allow the identification of the genomic make-up of tumours and provides a new means for
studying cancer patients in terms of monitoring tumour burden, assessing the mechanisms of
therapeutic response and resistance, detecting minimal residual disease, and understanding
unresolved biologic puzzles presented by tumour heterogeneity and clonal evolution (4-17).
We have reported a proof-of-principle study in the field of liquid biopsies, which is going
to be an ancillary article analysed in this thesis entitled: “Capturing intra-tumour genetic
heterogeneity by de novo mutation profiling of circulating cell-free tumour DNA: a proof-
of-principle” (10) published in Annals of Oncology in July 2014. This article is one of the first to
demonstrate that high-depth targeted massively parallel sequencing of plasma-derived ctDNA
constitutes a potential tool for de novo mutation identification and monitoring of somatic
genomic alterations during the course of targeted therapy, and this non-invasive tool may be
employed to overcome the challenges posed by tumour heterogeneity.