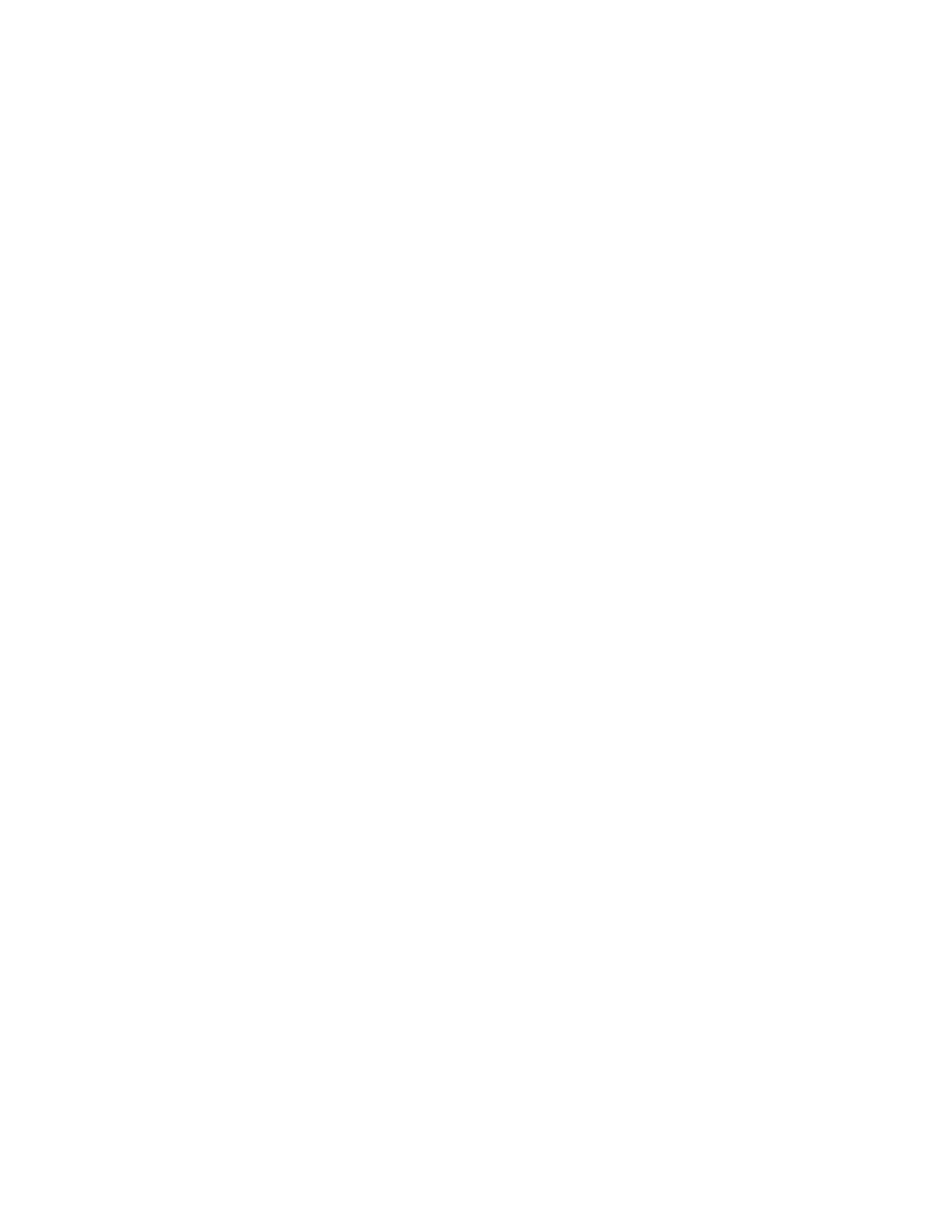
iii
Acknowledgements
I thank the Almighty God for making this work possible. A special thank you to Dr. D. Gwyn
Bebb under the tutelage of whom I carried out this project, thank you so much for accepting me
and given me the opportunity to practice science. It was quite a challenge but for your word of
encouragement which has kept me going from the beginning to the end; GOD BLESS YOU. To
my supervisory committee members, Drs. Sarah Childs and Susan Lees-Miller; I say a big thank
you. Dr. Lees-Miller, the project would not have been completed without your experience,
guidance and suggestions, I cannot stop thanking you for those prompt feedbacks on my work.
Dr. Childs, I’m really grateful for your keen scientific insights. To members of the Medical
Science department and especially Kiran Pandher, thank you all.
I want to extend my appreciations to my great teachers, Huong Muzik, Chris Williamson, Eiji
Kubota and Lars Petersen, for their technical knowledge and supports. Huong, I will forever
appreciate your patience and kindness. Eiji, I cannot thank you enough for your willingness to
always help out of your busy schedule, even while you are far away in Japan. Lars, I really
appreciate your efforts in making my work look professional and the time spent in reviewing my
write-ups.
To every member in Bebb’s lab, (especially Rachel, Shannon, Rosie, Allison,Will); Morris’s
Lab (especially Dr. Morris D., Qiao, Jason, Chandini); Lees-Miller’s lab; Translational Research
lab in general, (Brant, JB, Cay); Elaine Wilkie and everyone that has contributed positively to
my experience, I cannot name you all, thank you for making this a memorable experience.