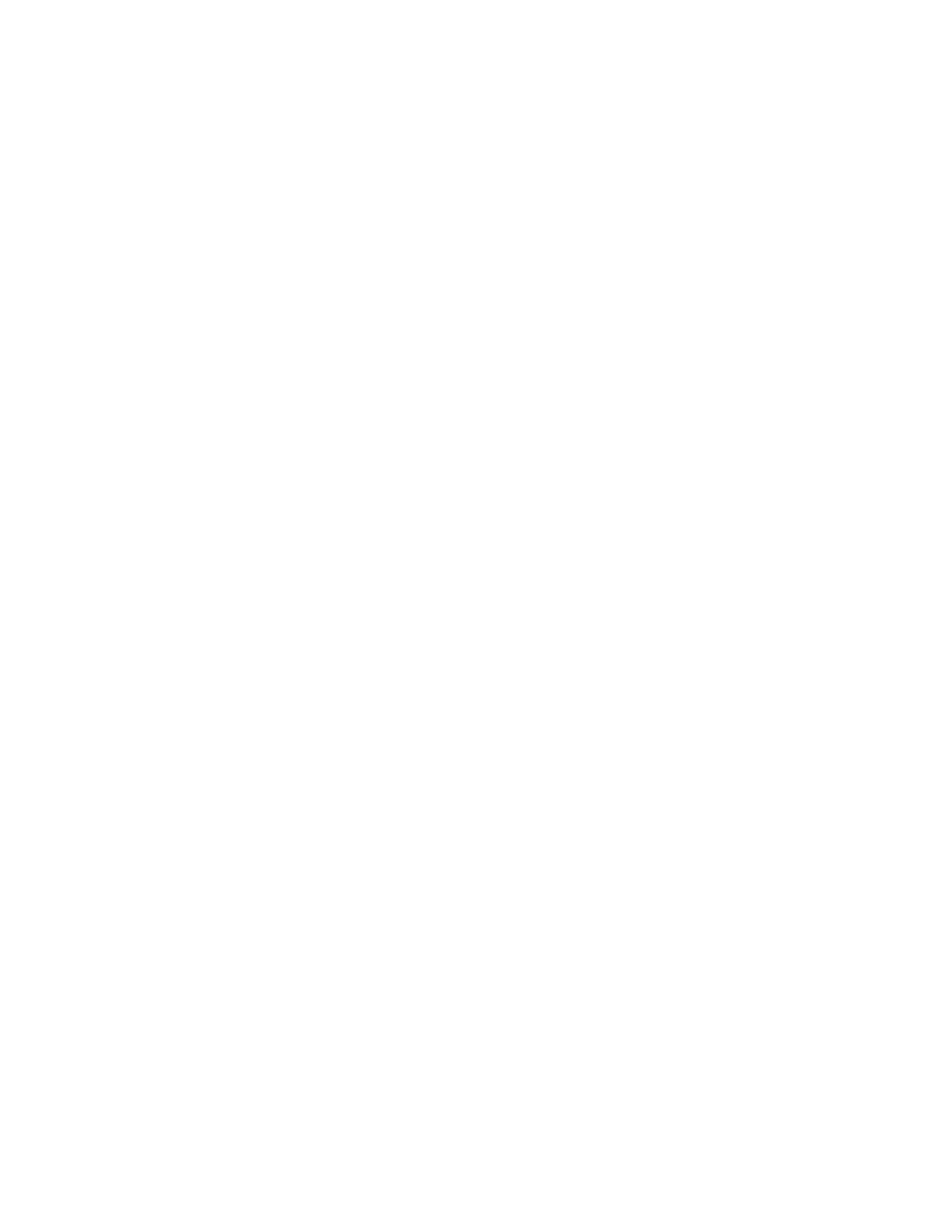
iii
Acknowledgements
My MSc studies would have not gone far without the invaluable support from a number
of individuals who I am very grateful for.
Most importantly, I would like to thank and acknowledge my supervisor Dr. Frank Jirik
for giving me opportunity to do research. I am very grateful for all of his support,
guidance, and encouragements. I have gained enormous amount of valuable information
from him and I will always have a great respect for him. His geniuses mind and passion
for science has always been inspiring and admirable.
I would like to thank my committee members Dr. Steve Boyd and Dr. Shirin Bonni. I am
very grateful for their supervision, kindness, and support. I will always appreciate their
time and helpfulness.
I am very appreciative for having a great support from all of the the Jirik lab’s member.
They have been very kind and supportive throughout the years I was in the lab. I would
like to send a special thanks to Jennifer Hahn who has been there for me as a wonderful
friend and an amazing guide throughout my master’s program. I really respect her work
ethics and happy to had the privilege to work alongside her. I would like to thank
Michelle Villemaire for her helpfulness, support, and friendship that help me to get
through some of the challenging days in my maters. I also like to thank Dr. Gary Martin
and Charlene Downey for their advice and help throughout my experiments. They are