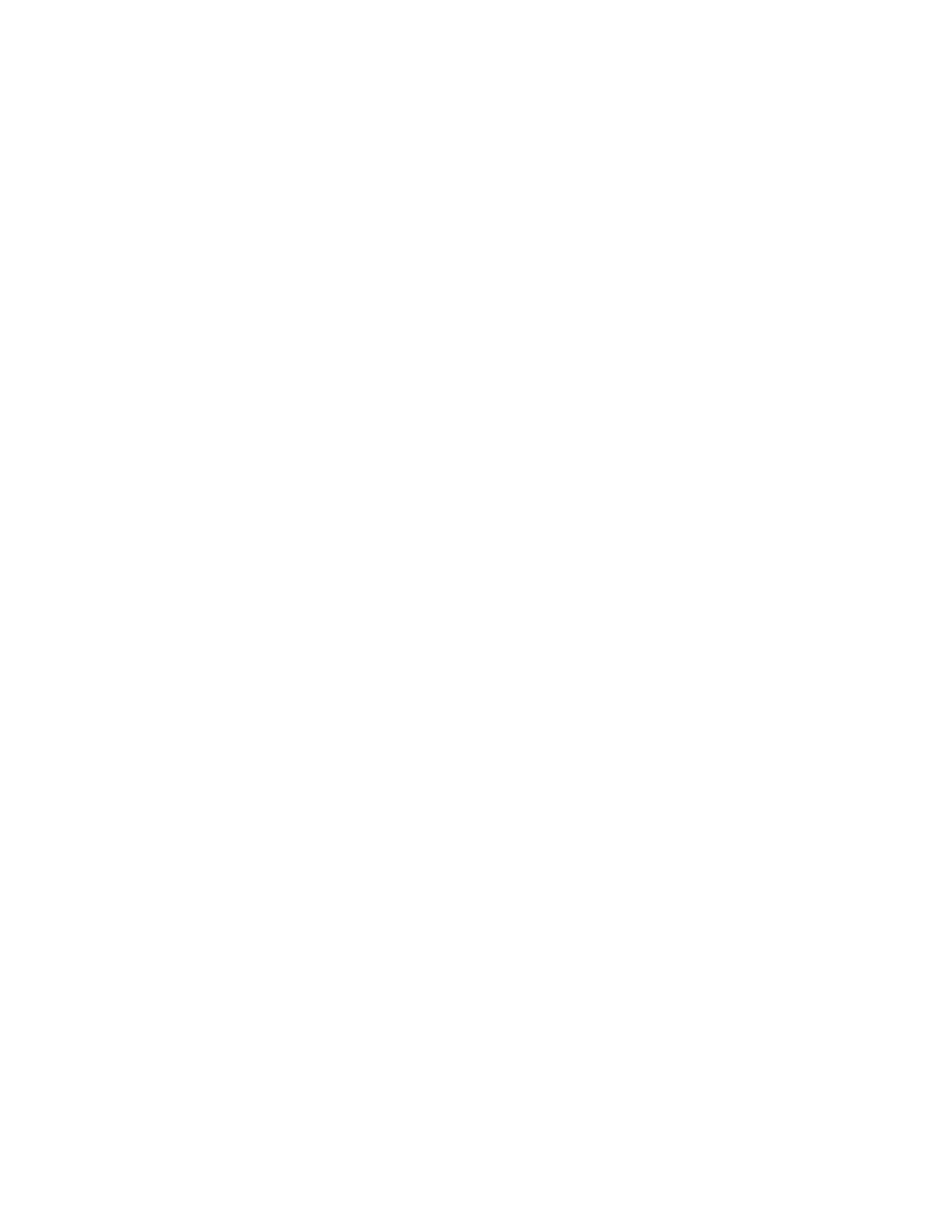
v
Table of Contents
Abstract ............................................................................................................................... ii!
Acknowledgements ............................................................................................................ iii!
Dedication .......................................................................................................................... iv!
Table of Contents .................................................................................................................v!
List of Tables ..................................................................................................................... ix!
List of Figures and Illustrations ...........................................................................................x!
List of Symbols, Abbreviations and Nomenclature .......................................................... xii!
Material & Methods ......................................................................................................... xiii!
Units of Measure .............................................................................................................. xiv!
CHAPTER ONE: INTRODUCTION ..................................................................................1!
1.1 Paediatric Cancer and Incidence ................................................................................1!
1.2 Embryonal tumors of childhood ................................................................................5!
1.2.1 Neuroblastoma ...................................................................................................6!
1.2.2 Atypical teratoid/rhabdoid tumor ......................................................................7!
1.3 Clinical aspects of NB and AT/RT ............................................................................8!
1.3.1 Age at diagnosis ................................................................................................9!
1.3.2 Tumor stage .......................................................................................................9!
1.3.3 Biological aspects ............................................................................................14!
1.3.3.1 Neuroblastoma .......................................................................................14!
1.3.3.2 Atypical teratoid/rhabdoid tumor ...........................................................16!
1.3.4 Therapeutic aspects .........................................................................................18!
1.3.4.1 Neuroblastoma .......................................................................................18!
1.3.4.2 Atypical teratoid/rhabdoid tumor ...........................................................19!
1.4 Role of Receptor Tyrosine Kinases in cancer ..........................................................21!
1.4.1 EGFR and its role in cancer .............................................................................22!
1.4.1.1 Targeting EGFR/HER-2 ........................................................................28!
1.4.2 IGF-1R and its role in Cancer .........................................................................29!
1.4.2.1 Targeting IGF-1R ..................................................................................32!
1.4.3 Role of serine/threonine kinases in cancer ......................................................36!
1.4.3.1 The PI3K pathway .................................................................................37!
1.4.3.2 The MAPK pathway ..............................................................................38!
1.4.4 Lapatinib as an EGFR/HER-2 inhibitor ..........................................................41!
1.4.5 Ponatinib as an IGF-1R inhibitor ....................................................................42!
1.4.6 Cobimetinib as an MEK1/2 inhibitor ..............................................................43!
1.4.7 Cancer cell migration and metastasis ..............................................................44!
1.5 Hypothesis ...............................................................................................................46!
1.5.1 Specific Aims ..................................................................................................46!
CHAPTER TWO: MATERIAL AND METHODS ..........................................................47!
2.1 Cell Culture ..............................................................................................................47!
2.1.1 Freezing and thawing of cells ..........................................................................47!
2.1.2 Passaging of cells ............................................................................................48!
2.2 Small molecule kinase inhibitors and chemotherapeutic agents .............................50!
2.3 Cell viability assays .................................................................................................50!