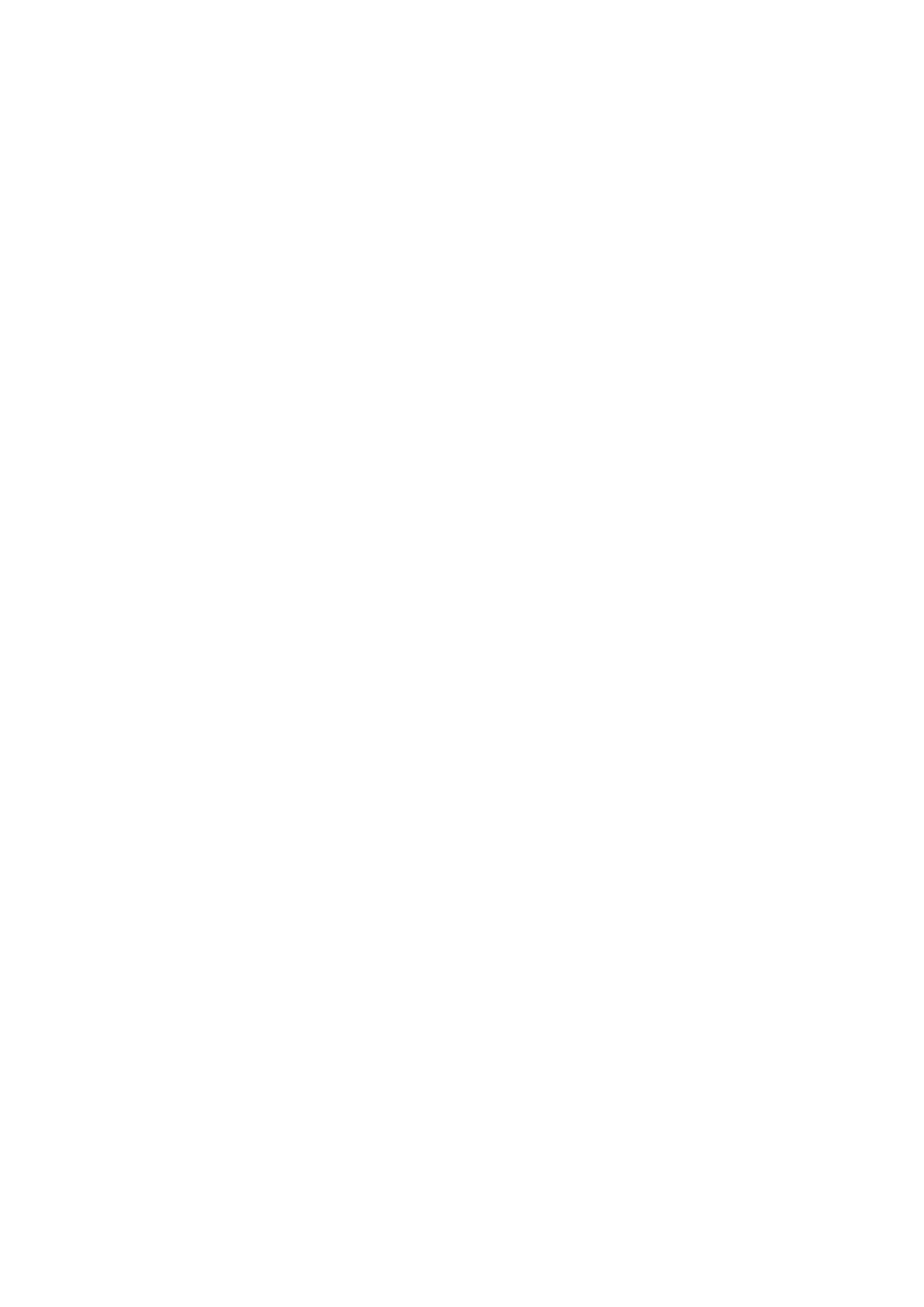
3
ACKNOWLEDGEMENTS
Firstly, I would like to express my sincere gratitude to my exceptional advisors
Dr. Laurence Huc and Dr. Fabrice Pierre for their continuous support for my PhD study and
related research, also for their patience, motivation and immense knowledge. Their guidance
helped me in all the time of research and writing of this thesis. I could not have imagined
having better advisors and mentors for my PhD study.
Besides my advisors, I would also like to thank the members of my thesis committee
and examination panel: Dr. Dominique Lagadic-Gossmann, Dr. Nicolas Loiseau,
Pr. Catherine Muller, Pr. Saadia Kerdine-Römer, Dr. Mojgan Djavaheri-Mergny and Dr.
Adrien Rossary, for their guidance, insightful comments, critical analyses and encouragement,
also for their questions which incented me to widen my research from various perspectives.
I thank all fellow labmates in Team E9 (Prevention and Promotion of Carcinogenesis
by Food) since my arrival in the team: Dr. Cécile Héliès-Toussaint, Dr. Françoise Guéraud,
Dr. Jacques Dupuy, Dr. Nathalie Naud, Dr. Sylviane Taché, Dr. Maryse Baradat, Dr. Sabine
Dalleau, Dr. Océane Martin, Dr. Natalie Priymenko, Dr. Vanessa Graillot, Dr. Julia Keller
and all the interns. In particular, I am grateful for Pr. Denis Corpet for supporting and
recommending me doing my internship and PhD study in this incredible team. My sincere
thanks also goes to Dr. Thierry Gauthier who helped me realize experiments related to
confocal microscopy in his sophisticated platform for the last three years.
Last but not the least, I would like to thank sincerely my family and friends, in France,
in Indonesia and all over the world, for supporting me spiritually throughout the realization of
this thesis and my life in general. I am really grateful to have you all in my life.