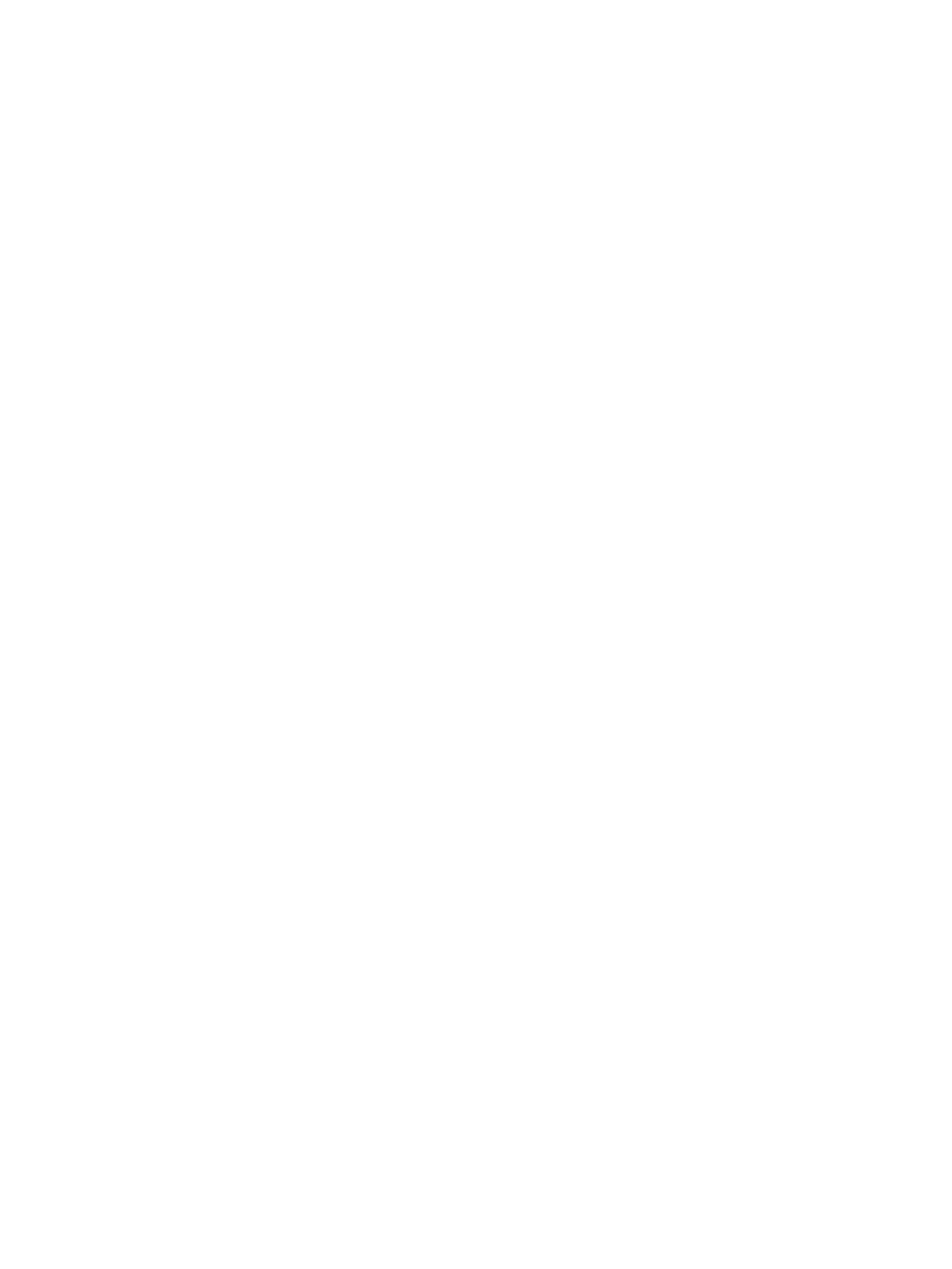
2.1. Angiogenesis in cancer
Angiogenesis is defined as the formation of new blood vessels from
existing ones. For solid tumors (1–2mm
3
), oxygen and nutrients can
reach the center of the tumor by simple diffusion. Because of their
non-functional or non-existent vasculature, non-angiogenic tumors
are highly dependent on their microenvironment for oxygen and the
supply of nutrients. When tumors reach 2 mm
3
, a state of cellular
hypoxia begins, initiating angiogenesis. Angiogenesis is regulated by a
fine balance of activators and inhibitors [11]. In the angiogenesis
process, five phases can be distinguished: 1. endothelial cell
activation, 2. basement membrane degradation, 3. endothelial cell
migration, 4. vessel formation, and 5. angiogenic remodeling. Hypoxia
increases cellular hypoxia inducible factor (HIF) transcription, leading
to upregulation of pro-angiogenic proteins such as vascular endothe-
lial growth factor (VEGF), platelet derived growth factor (PDGF) or
tumor necrosis factor-α(TNF-α)[12]. Activated endothelial cells
express the dimeric transmembrane integrin α
v
β
3
, which interacts
with extracellular matrix proteins (vibronectin, fibronectin, a.o.) and
regulates the migration of the endothelial cell through the extracel-
lular matrix during vessel formation [13]. The activated endothelial
cells synthesize proteolytic enzymes, such as matrix metalloprotei-
nases, used to degrade the basement membrane and the extracellular
matrix. The inner layer of endothelial cells undergoes apoptosis
leading to formation of the vessel lumen. Immature vasculature
undergoes extensive remodeling during which the vessels are
stabilized by pericytes and smooth-muscle cells. This step is often
incomplete resulting in irregular shaped, dilated and tortuous tumor
blood vessels [14]. This ability of tumors to progress from a non-
angiogenic to angiogenic phenotype (called the “angiogenic switch”)
is central to progression of cancer and allows the dissemination of
cancer cells throughout the body, leading to metastasis [11,15].
2.2. Enhanced Permeability and Retention (EPR) effect
Structural changes in vascular pathophysiology could provide
opportunities for the use of long-circulating particulate carrier
systems. The ability of vascular endothelium to present open
fenestrations was described for the sinus endothelium of the liver
[16], when the endothelium is perturbed by inflammatory process,
hypoxic areas of infracted myocardium [17] or in tumors [18]. More
particularly, tumor blood vessels are generally characterized by
abnormalities such as high proportion of proliferating endothelial
cells, pericyte deficiency and aberrant basement membrane formation
leading to an enhanced vascular permeability. Particles, such as
nanocarriers (in the size range of 20–200 nm), can extravasate and
accumulate inside the interstitial space. Endothelial pores have sizes
varying from 10 to 1000 nm [19]. Moreover, lymphatic vessels are
absent or non-functional in tumor which contributes to inefficient
drainage from the tumor tissue. Nanocarriers entered into the tumor
are not removed efficiently and are thus retained in the tumor. This
passive phenomenon has been called the “Enhanced Permeability and
Retention (EPR) effect,”discovered by Matsumura and Maeda [20–22].
The abnormal vascular architecture plays a major role for the EPR
effect in tumor for selective macromolecular drug targeting at tissue
level that can be summarized as follows and illustrated in Fig. 2:
(1) Extensive angiogenesis and hypervasculature
(2) Lack of smooth-muscle layer, pericytes
(3) Defective vascular architecture: fenestrations
(4) No constant blood flow and direction
(5) Inefficient lymphatic drainage that leads to enhanced retention
in the interstitium of tumors
(6) Slow venous return that leads to accumulation from the
interstitium of tumor
Physiological changes in blood flow within the tumors and in
transport properties of tumor vessels are consequences of these
vascular abnormalities. In 1987, Jain hypothesized that the osmotic
pressure in tumors must be high. This high tumor interstitial fluid
pressure (IFP) could be a barrier for efficient anti-cancer drug delivery
[23]. It is now well known that the IFP of most solid tumors is
increased. Many anti-cancer drugs —high molecular weight com-
pounds in particular —are transported from the circulatory system
through the interstitial space by convection rather than by diffusion.
Increased IFP contributes to a decreased transcapillary transport in
tumors, leading to a decreased uptake of drugs into tumor. In addition,
IFP tends to be higher at the center of solid tumors, diminishing
toward the periphery, creating a mass flow movement of fluid away
from the central region of tumor. To ensure that all the tumor get an
adequate drug supply, drug molecules or drug-loaded nanocarriers
should migrate through the tumor interstitial space from a site of
entry to remote cells. This process is hindered by high IFP. Due to their
greater size, the transport of drug-loading nanocarriers is less affected
by this enhanced IFP in tumors. Moreover, the microvasculature
pressure in tumors is also one to two orders of magnitude higher than
in normal tissues. This facilitates extravasation of nanocarriers that
could otherwise have been precluded by high IFP. Many types of
nanocarriers successfully overcome these barriers and selectively
accumulate in the tumors [23–25].
2.3. pH
While the intracellular pH of cells within healthy tissues and
tumors is similar, tumors exhibit a lower extracellular pH than normal
tissues. Accordingly, although tumor pH may vary according to the
tumor area, average extracellular tumor pH is between 6.0 and 7.0
whereas in normal tissues and blood, the extracellular pH of is around
7.4 [26,27]. Low pH and low pO
2
are intimately linked and a variety of
insights now support their roles in the progression of tumor from in
situ to invasive cancer [28]. The low extracellular tumor pH mostly
arises from the high glycolysis rate in hypoxic cancer cells. Amazingly,
this ATP-generating pathway is also exploited by tumor cells when
oxygen is available [29]. This phenomenon named the Warburg effect
emphasizes that proliferating tumor cells do not exploit the full
capacity of glucose oxidation to produce energy. Both defects in the
mitochondrial respiratory chain and the need of glycolysis-derived
biosynthetic intermediates account for this metabolic preference
(reviewed in [29]). To maintain a high glycolytic rate however requires
that pyruvate is converted into lactate to generate nicotinamide
adenine NAD+, a factor required by different glycolytic enzymes.
Lactate itself needs to be eliminated from the cell to favor the
metabolic flux and avoid cytotoxicity development. Monocarboxylate
transporter will export one proton together with one lactate molecule,
leading to a progressive acidification of the tumor extracellular space
(and a slight alkalinisation of the cytosol). Hypoxia-induced expres-
sion of carbonic anhydrase IX (CA IX) will also contribute to exacerbate
the pH gradient between the intra- and extracellular compartments
through the conversion of CO
2
to bicarbonate and subsequent uptake
of this weak base through the anion exchanger Cl-/bicarbonate [30].
The resulting pH gradients between intra- and extracellular tumor
cells but also between the tumor mass and the host tissue are
therefore potential sources of differential drug partitioning and
distribution. In a low pH extracellular environment, the uncharged
fraction of a weak acid increases and such a drug can thus more easily
diffuse through the cell membrane. The relatively basic intracellular
compartment may in turn favor the ionization of the molecule,
thereby promoting the cytosolic accumulation of the drug. Alteration
in this process is proposed to contribute to the multidrug resistance
(MDR) phenomenon [31]. The exposure to chemotherapy may indeed
favor the selection of tumor-cell clones with very acidic organelles
which will trap drugs and thereby reduce their activity; if this
137F. Danhier et al. / Journal of Controlled Release 148 (2010) 135–146