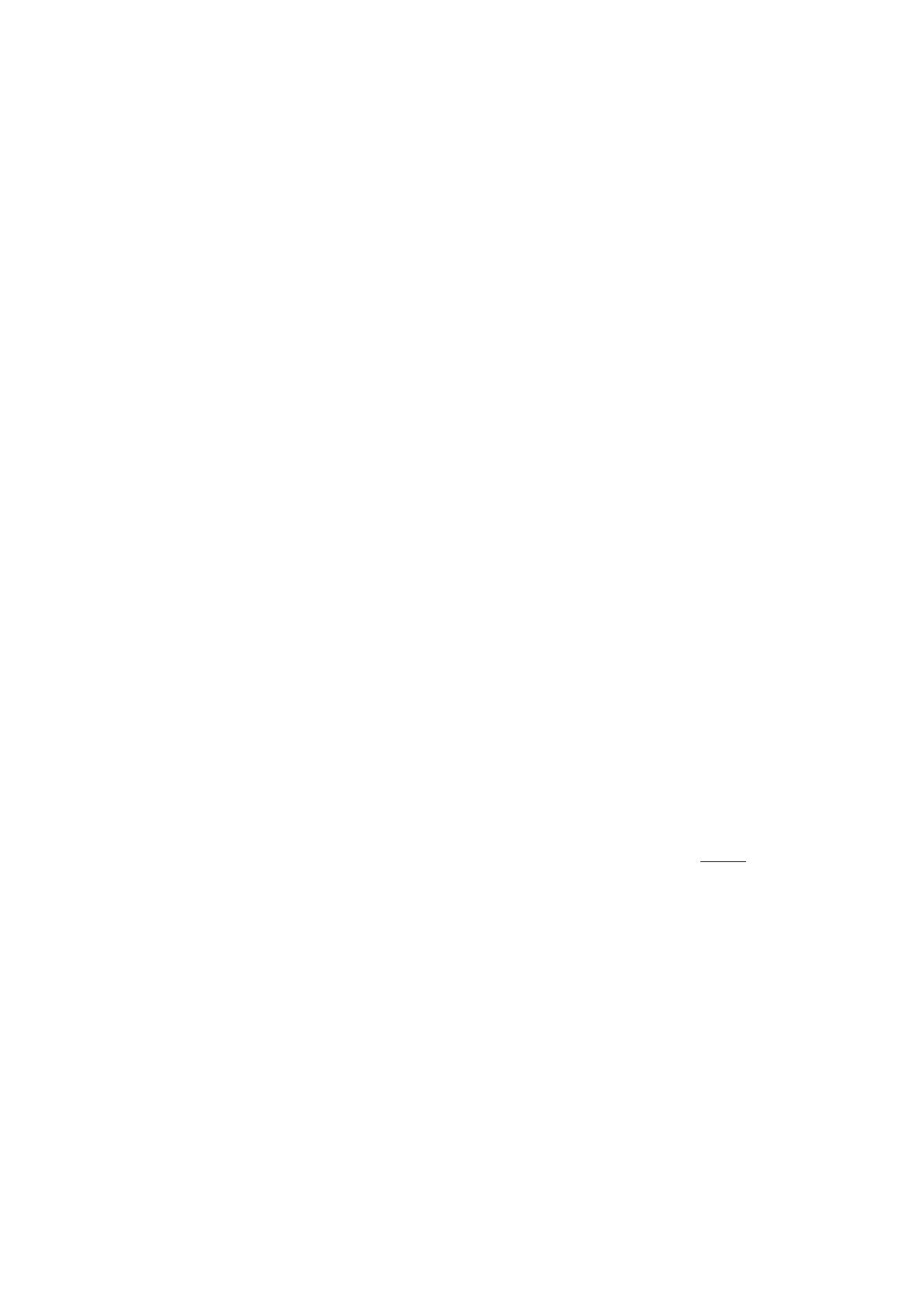
récent travail de DEA mais aussi pour sa gentillesse. Merci à la « toute petite dernière » arrivée, Christelle (de carnold gal) pour sa
sympathie et simplicité et bon courage pour la plate-forme, fais nous bien tourner ça ! Merci surtout de n’avoir pas craqué avec le live de
Noir Dés en boucle ! Plein de bonheur et de bons horoscopes pour la suite ! Merci à toi Sam pour ton amitié, ton goût pour la coinche, et ton
stage même s’il fut un peu court au final mais bon… on a quand même réussi ensemble à lancer la dernière ligne droite de ma thèse, à tenir
les délais et faire tout ce qui était prévu… et en plus ça t’a plu, cool! Merci Eric pour ton aide technique très précieuse durant ces derniers
mois de thèse. Merci Soraya pour la courte mais très plaisante (en plus d’efficace !) période de travail que nous avons déjà pu partager. Je
suis très heureux d’avoir pu ressentir ta motivation, rigueur et ton intérêt pour la science parce que ça m’a remis la pêche à un moment où
j’en avais besoin et te souhaite donc plein de réussite et du bonheur pour la suite ! Votre aide à tous les trois a été vraiment capitale pour que
je puisse boucler un maximum de projets presque à temps… donc, un vrai très grand merci à tous les 3 pour tout !!!
Finalement, un merci tout spécial à un membre passé mais encore présent mais presque passé quand même de l’équipe de
génétique humaine … re-… mais surtout et avant tout mon coloc’… Pixote, dit Vincent ou CarnacMan à l’occase. Pixxxote !! Merci, et pas
que pour le soutien ces derniers temps et surtout plein de courage pour bien redémarrer. C’est ta chance. Là bas. Il y a du thym, de la bruyère.
Tout est neuf et tout est sauvage. Et tout est possible à ton âge. Tout mais pas l’indifférence. Si tu as la force et la foi. Je ne vous parlerai pas
d’elle. Pas toi. Compte pas sur moi. J’accepterai la douleur. Que les vents te mènent où d'autres âmes plus belles sauront t'aimer mieux que
nous puisque l'on ne peut t'aimer plus. Je crois que j’ai trop veiller tard. Fatiguééé… voilà c’était ma ptite dédicace... puisque tu pars ! Même
si t’en as rien à secouer, je crois fortement en toi alors tache de pas me décevoir… j’ai parié des ronds.
Merci pour toutes les très très belles rencontres que cette thèse m’a permis de faire dans le labo 3045 et qu’on a ensemble bien
vite emmené au-delà du professionnel :
- et tout d’abord merci aux deux compères de la première heure, Krishna et Richard, exilés depuis bien trop longtemps du labo:
Krishna, je passe très rapidement sur la qualité irréprochable de ton travail en toutes circonstances mais ce pour te dire que je te remercie
très sincèrement pour tout ce que tu m’as appris et as fait pour moi. Et surtout pour l’ami que tu es, tu sais combien je t’adore ! Bonne
continuation à Londres ou ailleurs et qu’on arrive à se capter un peu plus ! May the force of winds and zouklove stay in you forever !
Richard, ben je crois qu’on se connaît suffisamment tous les 2 pour pouvoir t’annoncer que je vais juste te cirer les pompes vite fait bien
fait, simplement parce que je sais qu’il y a d’autres personnes qui liront ce paragraphe donc j’y suis un peu obligé ☺… Je voudrai donc
d’abord te remercier au niveau boulot… tout simplement parce que si tu n’étais pas « passé » avant moi, je n’aurai simplement certainement
pas pu faire tout ce que j’ai fait. Loin de là. Merci aussi d’avoir été présent et à l’écoute ce fameux soir de résultats de DEA où j’ai
finalement décidé de pas tout envoyer péter tout de suite et de faire cette thèse. Sans doute un tournant. Les deux années partagées ensemble
au labo ont été plus que sympas. Une spéciale dédicace pour une de mes (nos même je crois) blagues préférées (sic) : merci aux puces
pangénomes in France qui devaient arriver bien avant la fin de ta thèse et qui sont finalement arrivées tout juste avant la fin de la mienne (re-
sic)… Tout aussi sérieusement, merci de partager fortement certaines convictions sur ce boulot. Une dédicace aux scientifiques qui
mélangent à notre goût un peu trop intérêts personnels, science et politique ? No way ! Enfin, le plus important pour moi, un vrai merci très
perso pour la belle amitié qu’on a pu nouer à Strasbourg et même si on est plus éloigné aujourd’hui, je voulais te dire que le ptit jaune est
aussi bon sur votre gazon anglais que sur votre ex-terrasse strasbourgeoise… gageons de voir quel goût il aura sur les bord du Léman…
rendez vous est pris donc ! Une très très grosse bise à Aude au passage.
- merci ensuite à mes voisins directs de labo qui ont grandement contribué à améliorer mon environnement quotidien rien que par leur
présence (enfin ça, ça dépend des jours)… et qui sont également devenus de très grands amis :
Merci à toi Fabrice… dit Faby ou encore le Fab, pour tes extraordinaires compétences de bioch et de bio structurale… nan, ça c’était pour
rire. J’ai vraiment beaucoup apprécié et continue heureusement à pouvoir le faire, nos nombreux échanges de ces dernières années et en
particulier les apéros, discussions, bouffes nocturnes ou week-ends en tous genres… Longue vie aux sound-systems dans les labos, aux
fraises-planètes et à tes trop nombreuses dents ;o) ... mois aussi je t’M. A bientôt pour un nouveau pèlerinage en terre sainte ?
La meilleure (ouah !!!!) des 3045 (oooooh) pour la fin (haaaaaaaannnnnnnnn lala… dis moi pas ☺ ) … je te remercie Marie pour ces années
passées côte à côte, ou plutôt dos à dos (enfin ces derniers temps, je préfère ça que ventrre à ventre ;o). Merci, d’abord parce que tu m’as
appris beaucoup beaucoup… sur moi, sur les autres et aussi sur moi et les autres… Merci, ensuite, pour toutes les fois où tu m’as soutenu,
voire simplement tenu, moralement. Merci enfin d’être l’amie que tu es. In case you’d encounter any trouble water in the future… Bon
courage pour les mois/jours qu’il te reste à être un peu grosse et surtout ne bouge pas, c’est un ordre ;o) !
Merci à toi Schkoumy™ (et oui !... c’est moi qui l’a trouvé… et c’est comme ça que ça s’écrit !)… dit Schkoum’, Twix, Raie-
man, Café ? ou encore Stef. Même si on a mis un peu de temps au départ, je crois, et de l’avis de tout le monde, que les 2 Twix se sont bien
trouvés dans une période où nos vies respectives changeaient quand même pas mal. Bref, merci pour tout ce qu’on a vécu jusqu’à présent: les
apéros partagés sur les terrasses ou à domicile jusqu’à des pas d’heure, les fou-rires, les breakages, tes super bouffes, les nanas (ah non… ça
on a pas fait encore), la piscine, les coinches, le prix de la base et autres débats acharnés…. De toutes façons, ne t’inquiètes pas, tu sais bien
que « Demain…. Demain, il fera beau… sur le chemin… Manana…. Manana, es sera bien en el camino » ©Manu, Barcelone, Novembre
2004… et je rajouterais : « ok… mais con la ballelleta en el camino alors ».
Gégé, je te remercie du fond du cœur : je suis vraiment très heureux qu’on ait partagé nos vies pour faire un bon bout de chemin
et grandir ensemble. On a beaucoup appris tous les deux et j’espère sincèrement qu’on ne se perdra pas trop à l’avenir.
Merci à tous les potes avec qui on a partagé de tellement bons moments durant ces années. Un clin d’œil spécialement amusé à
toi Lolo… pour m’avoir mis une pression extérieure et continuelle mais salutaire pour que je « soigne le cancer »… ;o). Et à toi François
pas que pour les quelques dépannages de ces dernières semaines.
Merci enfin à Félix Gray et Didier Barbelivien… et à toutes les filles qu’on a aimées avant.
Un énorme Merci à l’ensemble de ma famille… bonne nouvelle, ça y est presque, je vais bientôt plus être étudiant et ptêt même
que je vais avoir un vrai « travail »… Plus spécialement, des grosses bises à ma grand-mère, mes parents et mes deux ptits frangins pour
leur soutien et leur présence depuis que je suis loin. Pour l’heureux Papa, désolé d’avance mais je n’ai pas pu résister… comme je te l’ai déjà
dit, docteur c’est sûr c’est pas ingénieur… mais c’est pas mal aussi ;o) (oh ça va c’était pour rire !). Benj et Lucien, que du bonheur et le
meilleur pour tout ce qui va venir !
Merci à Carole… pour Tout., mais surtout d’avoir accepté de ne partager que des rares bouts de week-end ces derniers mois.
Merci à celles et ceux que j’aurai bien involontairement oubliés…