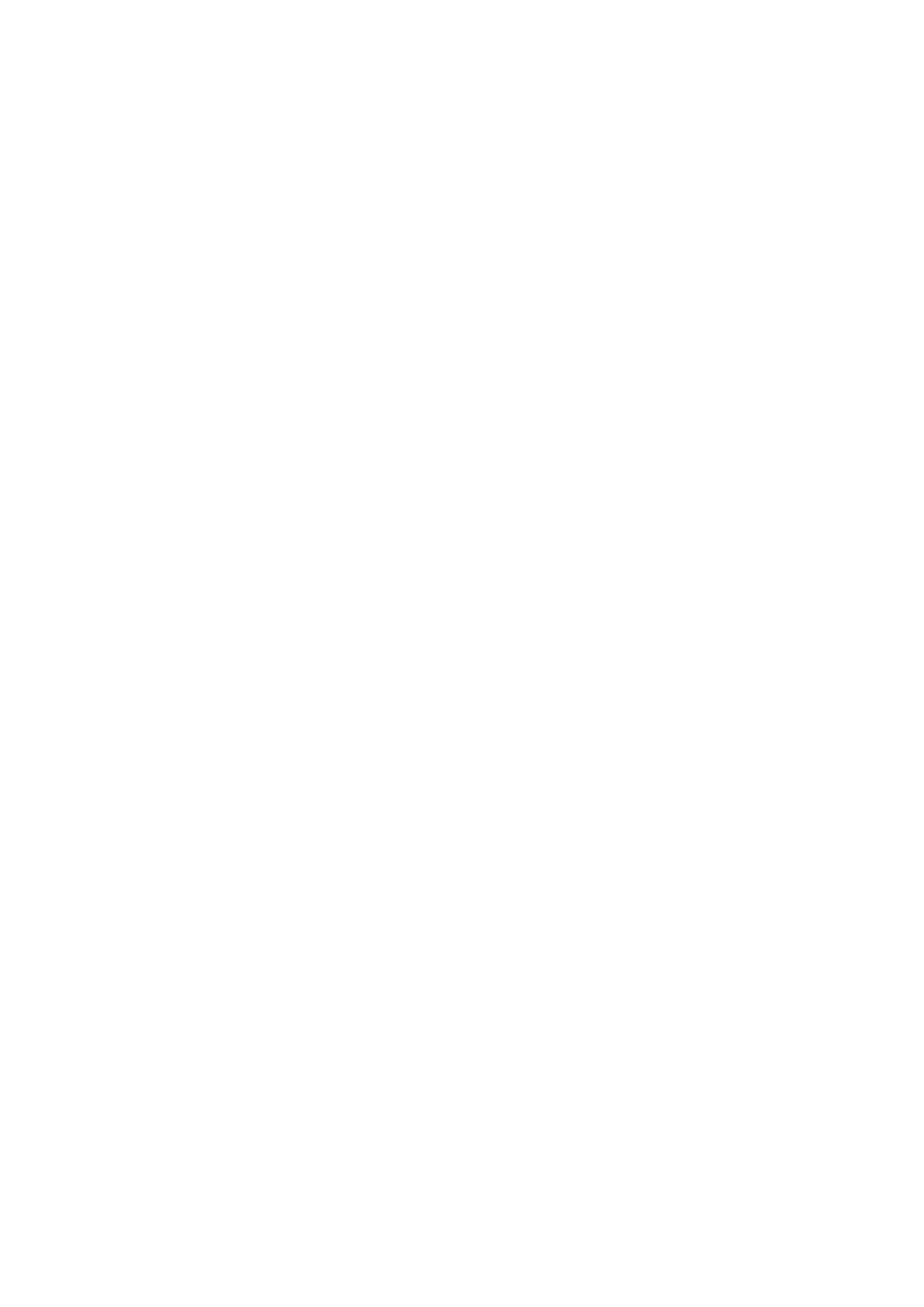
LES P’TITS MOTS DE P’TITE CAROLE
Eh bien nous y voilà, il est temps pour moi de vous écrire quelques mots… J’ai
repoussé ce moment au maximum, car cela représente la fin d’un chapitre de ma vie et
j’avoue que j’ai du mal à l’accepter !
Marc, notre grand manitou, je te remercie de m’avoir accueillie dans ton équipe
depuis mon stage de master 2, même si à cette époque tu avais dit ‟on ne prend plus de filles
dans le labo”… ! J’espère que tu ne regrettes pas ! En tous cas merci de m’avoir donné
cette chance de travailler sur ce sujet si passionnant. Je souhaite vraiment que ta motivation
durant toutes ces années permette à OncoVita de voir le jour. Ton emploi du temps de
ministre m’a toujours impressionnée et tu vas maintenant devenir directeur de l’unité mais
j’espère que tu pourras toujours te dégager du temps pour endosser ta casquette de ‟papy
gâteau” ! Je n’oublierai pas ton sourire, ta générosité, ta musique zen et tes petites lunettes
rondes !
Jeff, mon directeur de thèse, je te dois un grand merci pour m’avoir encadrée toutes
ces années ! J’ai énormément apprécié tous les moments que nous avons passés à échanger,
j’ai beaucoup appris de toi grâce à ta motivation à me faire partager tes connaissances
scientifiques et ton expérience. Merci pour ta disponibilité face à mes questions incessantes,
mes doutes, mais aussi pour une dernière répétition de ma présentation pour Ottawa un
samedi matin en plein mois de juillet et pour tes réponses à mes appels de panique pendant ma
rédaction de thèse…! Ce long chemin qui est celui de réaliser une thèse a été une très bonne
expérience pour moi, très enrichissante, et ton enthousiasme face aux résultats a été porteur.
Garde cette passion qui t’anime ! Je te remercie pour ta rigueur dans la rédaction des
papiers et la réalisation des figures, cela a été très formateur pour moi.
J’ai été ravie d’aller avec toi au congrès OVC à Boston ! C’était vraiment très
intéressant et motivant, et nous avons passé de bons moments avec Johann, Cécile et Philippe
de Transgene. C’était en plus la première fois que je traversais l’Atlantique ! Le retour a été
moins drôle, surtout pour toi avec ton siège d’avion défraîchi et les petits voisins pas très
sages assis derrière toi… ! Sans oublier la course dans l’aéroport pour finalement rater
notre avion pour rentrer à Nantes…!
Je me souviendrai aussi toujours lorsque tu m’as annoncé qu’on irait au congrès DC
2014… Tu as commencé par me dire que tu étais allé à ce congrès en Australie, aux USA, en