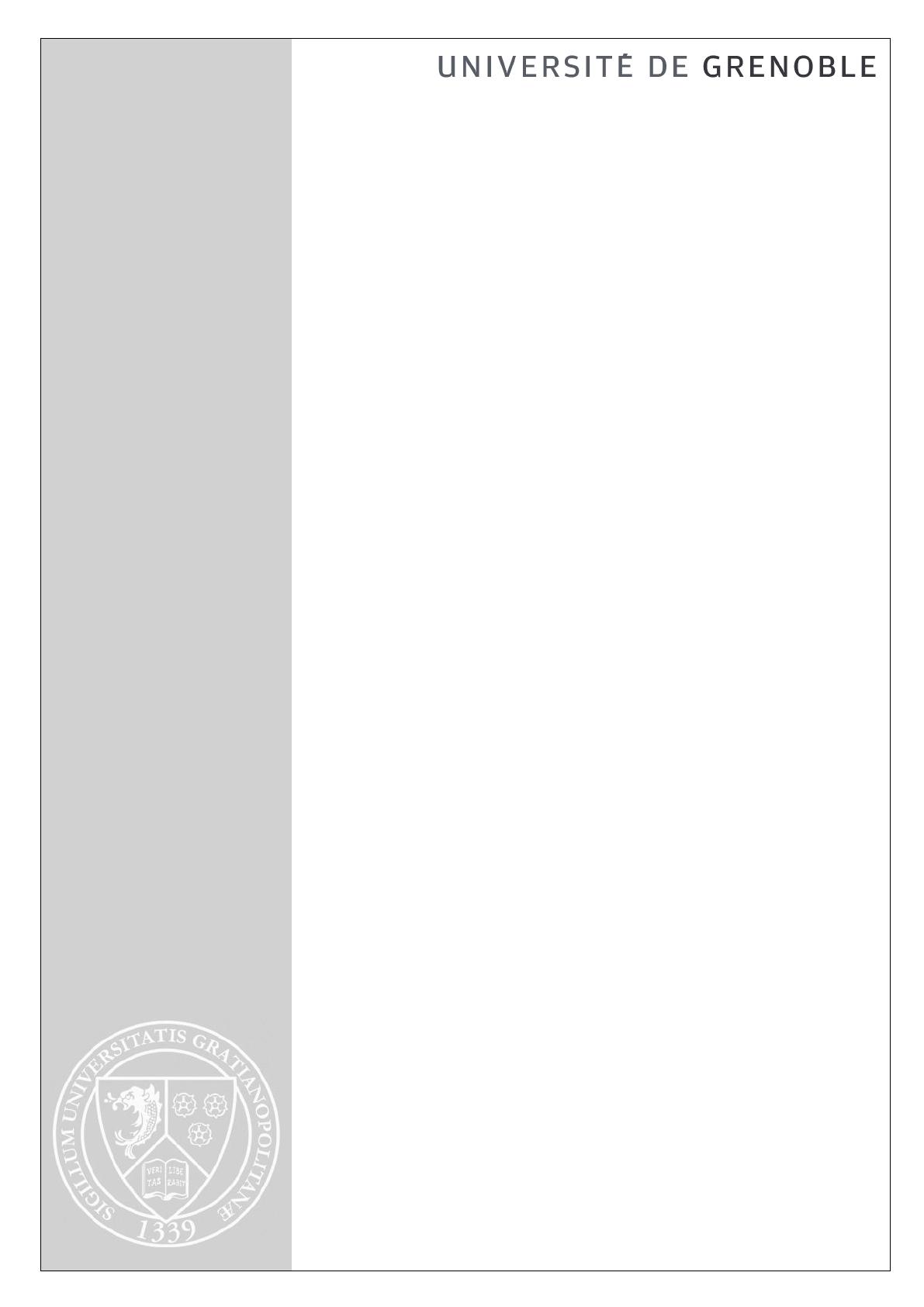
THÈSE
Pour obtenir le grade de
DOCTEUR DE L’UNIVERSITÉ DE GRENOBLE
Spécialité : Astronomie & Astrophysique
Arrêté ministériel : 7 août 2006
Présentée par
Julien Rameau
Thèse dirigée par Dr. Anne-Marie Lagrange
et codirigée par Dr. Gaël Chauvin
préparée au sein de l’Institut de Planétologie et d’Astrophysique de
Grenoble
et de l’Ecole doctorale de Physique de Grenoble
Caractérisation de la population de
planètes géantes à grandes sépa-
rations
Imagerie différentielle avec NaCo et SPHERE
au VLT
Thèse soutenue publiquement le 2 octobre 2014,
devant le jury composé de :
Pr., Claudine Kahane
Professeure, Université de Grenoble (France), Présidente
Dr., Claire Moutou
Directrice de recherche, Laboratoire d’Astrophysique de Marseille (France),
Rapporteur
Pr., René Doyon
Professeur, Université de Montréal (Canada), Rapporteur
Pr., Michael R. Meyer
Professeur, Ecole Polytechnique Fédérale de Zurich (Suisse), Examinateur
Dr., Christoph Mordasini
Chercheur, Institut d’Astronomie du Max Planck (Allemagne), Examinateur
Dr., Anthony Boccaletti
Chargé de recherche, Observatoire de Meudon/LESIA (France), Examinateur
Dr., Anne-Marie Lagrange
Directrice de recherche, Institut de Planétologie et d’Astrophysique de Grenoble
(France), Directrice de thèse
Dr., Gaël Chauvin
Chargé de recherche, Institut de Planétologie et d’Astrophysique de Grenoble
(France), Co-Directeur de thèse