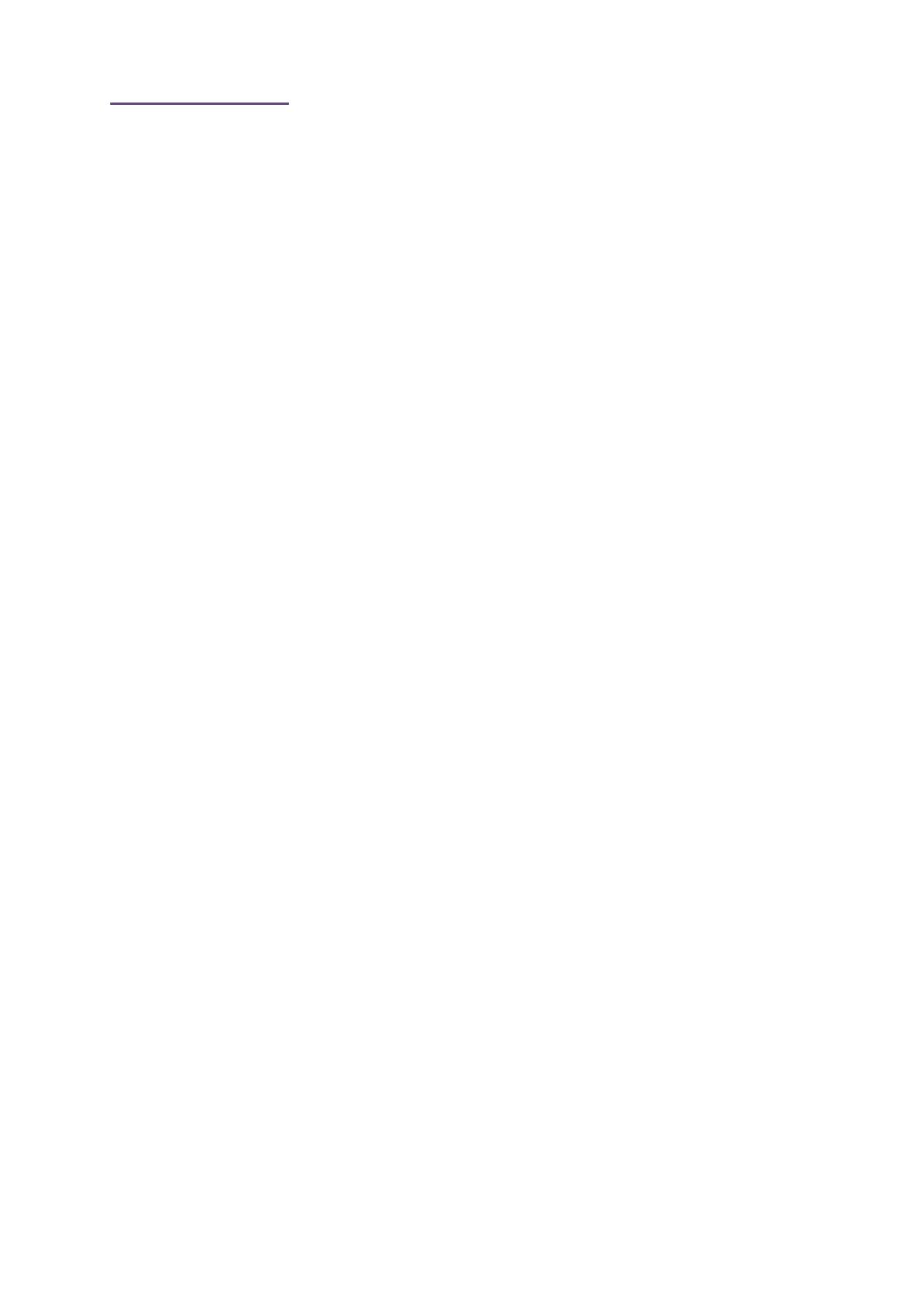
Remerciements :
Cette thèse est le fruit de quatre années de travail au sein de l’Institut de Neurobiologie de la
Méditerranée. Je remercie en premier lieu le Pr. Ben-Ari d’avoir fondé un tel laboratoire qui m’a
permis de travailler dans d’excellentes conditions tant au niveau scientifique qu’humain.
Je tiens ensuite à remercier tous les membres du jury : Les Drs Christine Métin et Antoine Depaulis
qui me font l’honneur d’être rapporteurs de ce travail, ainsi que les Drs Patrick Collombat et Harold
Cremer d’avoir accepté d’être examinateurs. Je remercie chaleureusement le Pr. André Nieoullon
d’avoir accepté de présider cette soutenance.
Je souhaite plus particulièrement remercier Alfonso Represa, mon directeur de thèse et directeur du
laboratoire, pour sa gentillesse, sa bonne humeur, sa disponibilité malgré son emploi du temps bien
chargé. Ses conseils, ses qualités scientifiques, et l’autonomie qu’il m’a laissé m’ont permis de grandir
et de m’épanouir scientifiquement.
Merci à tous les membres de l’équipe Represa et plus particulièrement Isabel pour avoir aider « la
petite » à ses débuts, Hélène pour toutes ces nombreuses heures passées à électroporer, Carlos pour
son aide précieuse en biologie moléculaire et Jean-Bernard pour nos discussions scientifiques et non
scientifiques ! Merci également à tous les membres de l’INMED qui ont contribué de près ou de loin à
cet environnement scientifique et humain très agréable, et plus particulièrement Valérie et Laurent
pour leur contribution électrophysiologique dans mon projet, et mon « roomate » préféré.
Une petite pensée pour « l’INMED team » sans qui ces quatre années n’auraient pas été aussi
agréables : Karine, Thibault, Belkacem, Bahija, Anice, Jennifer, Fred…Avec une mention spéciale aux
greluches : Perrinouche, Fabounette et Annele ! Une petite pensée aussi pour ma petite escalope qui
est toujours là à mes côtés depuis toutes ces années, 19 ans déjà !
Un grand merci à mes parents d’être toujours présents pour moi. C’est aussi grâce à eux que j’en suis
arrivée là, ils m’ont toujours épaulé et soutenu dans mes choix. Merci aussi à mes frères et mes belles
sœurs qui font de mon environnement familial un vrai bonheur ! Une petite pensée aussi pour tous
leurs petits bouts qui j’espère seront fiers de leur tata !
Je ne peux que finir ces remerciements par Laurent, qui me supporte quotidiennement, qui m’a aidé à
me défouler, quand la science n’avançait pas, pendant ces longues soirées de travaux, qui m’épaule au
quotidien, me réconforte, et qui est toujours là pour moi. Un grand merci à toi…