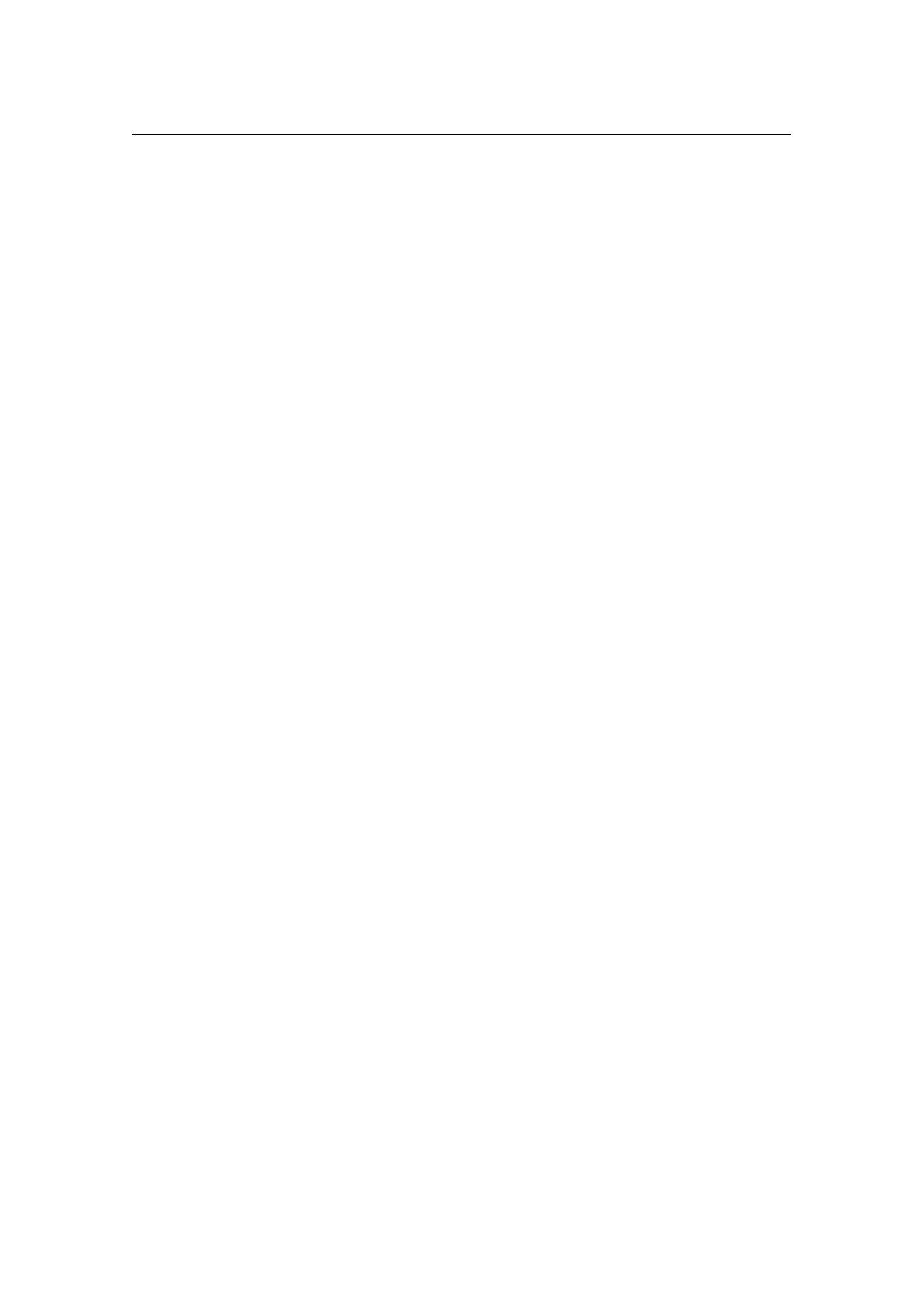
ii Contents
1.9 LaTiO3: structure and phase diagram .................... 14
2 Experimental set-up 17
2.1 Thin film deposition ............................. 17
2.1.1 Sputtering .............................. 18
2.1.2 Molecular Beam Epitaxy ...................... 19
2.2 Structural analysis of thin films ....................... 20
2.2.1 X-ray analyses ........................... 20
2.2.2 Transmission Electron Microscopy ................ 22
2.3 Lithographic patterning ........................... 23
2.4 Transport measurements: resistivity and Hall effect ............ 23
3 Ferroelectric Field Effect in thin superconducting films 25
3.1 Ferroelectric Field Effect .......................... 25
3.2 High-Temperature Superconductivity .................... 26
3.2.1 The polaron-bipolaron model ................... 27
3.2.2 The spin fluctuation scenario .................... 28
3.3 Ferroelectricity ............................... 29
3.3.1 Sawyer-Tower circuit ........................ 30
3.4 Ferroelectric Field Effect Devices ..................... 31
3.5 Thin Film Growth and Characterization .................. 33
3.5.1 Cuprate growth ........................... 33
3.5.2 Finite size effect in High-Tcthin films ............... 34
3.5.3 PZT thin films ........................... 37
3.6 Modulation of Superconductivity ...................... 39
3.6.1 PZT/GBCO/PBCO heterostructures ................ 39
3.6.2 P−Eand R−Eloops ...................... 39
3.6.3 Shift of Tc.............................. 40
3.6.4 Superconductor-insulator transition ................ 42
3.7 Modulation of Normal State Properties ................... 48
3.7.1 PZT/NBCO heterostructures .................... 48