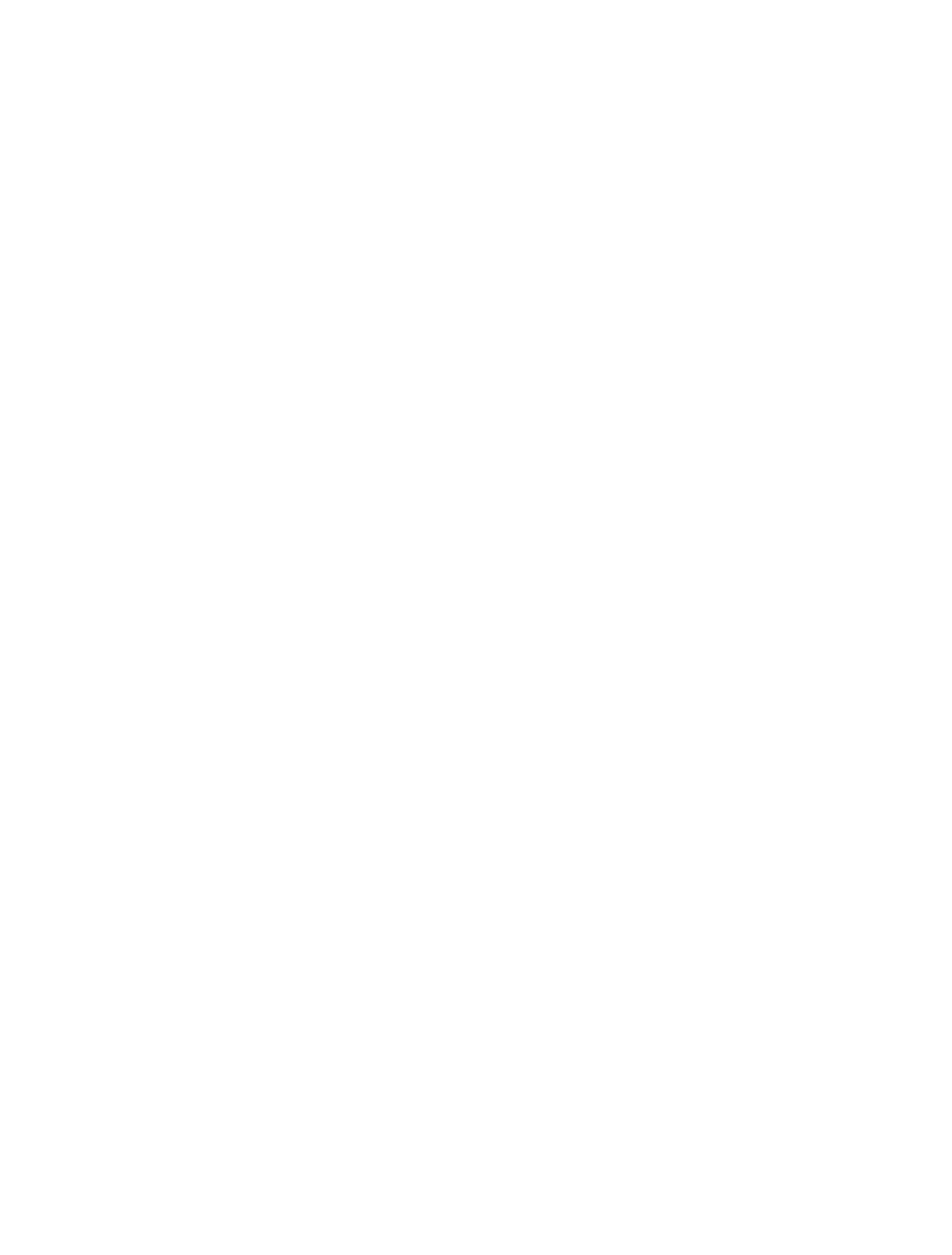
2386 Z. Sala et al.: Simulation of fragmental rockfalls detected using terrestrial laser scans
in the study of landslide hazards like rockfall (Jaboyedoff
et al., 2012). Terrestrial laser scanning (TLS) in particular
has been increasingly applied in recent years to the study
of rock slope instabilities (Abellán et al., 2014; e.g. Lato et
al., 2009, 2015; Kromer et al., 2017). TLS provides a de-
tailed 3-D model of the slope geometry, supporting a range
of geotechnical and geomechanical analyses. From a single
scan we can extract slope angle measurements, map rock
mass structure and look for evidence of past rockfall (Telling
et al., 2017). Using multiple successive scans of the same
slope, progressive changes to the slope surface can be mea-
sured. Multi-temporal scanning enables researchers to de-
tect rockfall events over time and build detailed magnitude–
frequency relationships for slopes, supporting the study of
processes such as coastal cliff erosion (e.g. Rosser et al.,
2007; Williams et al., 2018), as well as hazard and risk
assessments for linear infrastructure such as railways (e.g.
van Veen et al., 2017). The identification of rockfall events
using sequential scans also permits the back analysis of
rockfall-triggering factors and failure mechanisms. A study
by Kromer et al. (2015) used TLS change detection to in-
vestigate the occurrence of a 2600 m3failure above a section
of the Canadian National (CN) Railway in western Canada,
including an analysis of structural constraints, pre-failure de-
formation, and precursor rockfall leading up to failure.
While the application of TLS to rock slope monitoring is
often focused on determining how likely future rockfall is or
where the rockfall may come from, it is also important to de-
termine how likely it is that the rockfall material will reach
an element at risk should a fall occur. In order to answer that
question, rockfall modelling programs can be used to simu-
late the runout of falling rock material using numerical mod-
els (Turner and Duffy, 2012). A rockfall simulation requires
a representation of the slope surface and rockfall mass being
modelled in 2-D, 2.5-D, or 3-D. TLS can support rockfall
simulation by providing a high-resolution 3-D model of the
slope surface and, in cases where a rockfall event has been
identified using change detection between multiple scans, the
volume and location of the rockfall mass. In order to make
use of the quality and quantity of 3-D point cloud data be-
ing collected as part of a rock slope monitoring program
in western Canada, a 3-D rockfall simulation technique us-
ing game-engine technology has been developed (Ondercin,
2016; Sala, 2018). The technique uses the video game devel-
opment platform Unity 3D (Unity Technologies, 2018) and is
capable of simulating rockfall runout using fully 3-D meshes
built from TLS point cloud data.
One of the strengths of this simulation technique is its abil-
ity to simulate rockfall runout using numerous interacting
bodies. This capability allows us to produce simulations of
fragmental rockfall events, which are defined by the presence
of multiple mobile fragments of rock during runout (Hungr
and Evans, 1988). Conventional rockfall modelling programs
(e.g. RocFall, Rocscience Technologies, 2016; Rockyfor3D,
Dorren, 2015; RAMMS:ROCKFALL, Bartelt et al., 2016)
simulate single boulder trajectories at a time and there-
fore are unable to model fragmental rockfall runout. Efforts
to model fragmental rockfall processes, including the dis-
aggregation of falling rock masses along pre-existing dis-
continuities, or the breakage of intact blocks during im-
pact, have been demonstrated by previous authors. Cuervo
et al. (2015) utilized a discrete element technique to model
the disaggregation of a 1000 m3rockfall event in southern
France comprised of numerous mobile fragments. Wang and
Tonon (2011) developed a discrete element code which mod-
els the fragmentation of a falling rock at impact, taking into
consideration the effect of impact velocity, ground condition,
energy loss, and fracture properties such as persistence. The
GIS-based tool RockGIS, presented in Matas et al. (2017),
incorporates both breakage along pre-existing discontinuities
and the generation of new fragments during impact into a 3-
D runout modelling program and has been utilized to model a
10 000 m3fragmental rockfall in the eastern Pyrenees moun-
tains. The goal of the work presented in this paper is to
demonstrate the capability of our novel game-engine-hosted
simulation technique to model a series of rockfall events
detected using TLS change detection at two rock slopes in
south-central British Columbia. Emphasis is placed on the
ability of the technique to be used for fragmental rockfall
runout simulation, including a discussion of how these types
of simulation could support mitigation design.
2 Study sites
The rock slopes of focus for this paper are part of the
Ashcroft subdivision of the CN Railway. The subdivision
follows sections of the Thompson and Fraser River valleys,
located between the towns of Ashcroft and Lytton, British
Columbia. This region serves as an important transporta-
tion corridor for Canada, with the presence of the CN Rail
mainline, as well as sections of the Canadian Pacific Rail-
way, and the Trans-Canada Highway. A previous study by
Piteau (1977) identified that rock cuts in this region are sub-
ject to slope instability issues due to a combination of lat-
eral erosion from river activity, over-steepening from blast-
ing during the construction of the railways, and a lack of ad-
equate rockfall catchment areas.
Two sites in the Ashcroft subdivision will form the basis
for this research, White Canyon and Goldpan. The location
of these sites along the Thompson River and proximity to the
town of Lytton, BC, can be seen in Fig. 1. The monitoring of
these slopes is part of the Railway Ground Hazard Research
Program, a collaborative research initiative focused on the
characterization and assessment of mass movement hazards
affecting Canadian railways.
Data collection in the Ashcroft subdivision first took place
in 2012, and regular monitoring at both sites has been ongo-
ing since 2014, with data collection taking place on a sea-
sonal basis, approximately every 3 months. Point cloud data
Nat. Hazards Earth Syst. Sci., 19, 2385–2404, 2019 www.nat-hazards-earth-syst-sci.net/19/2385/2019/