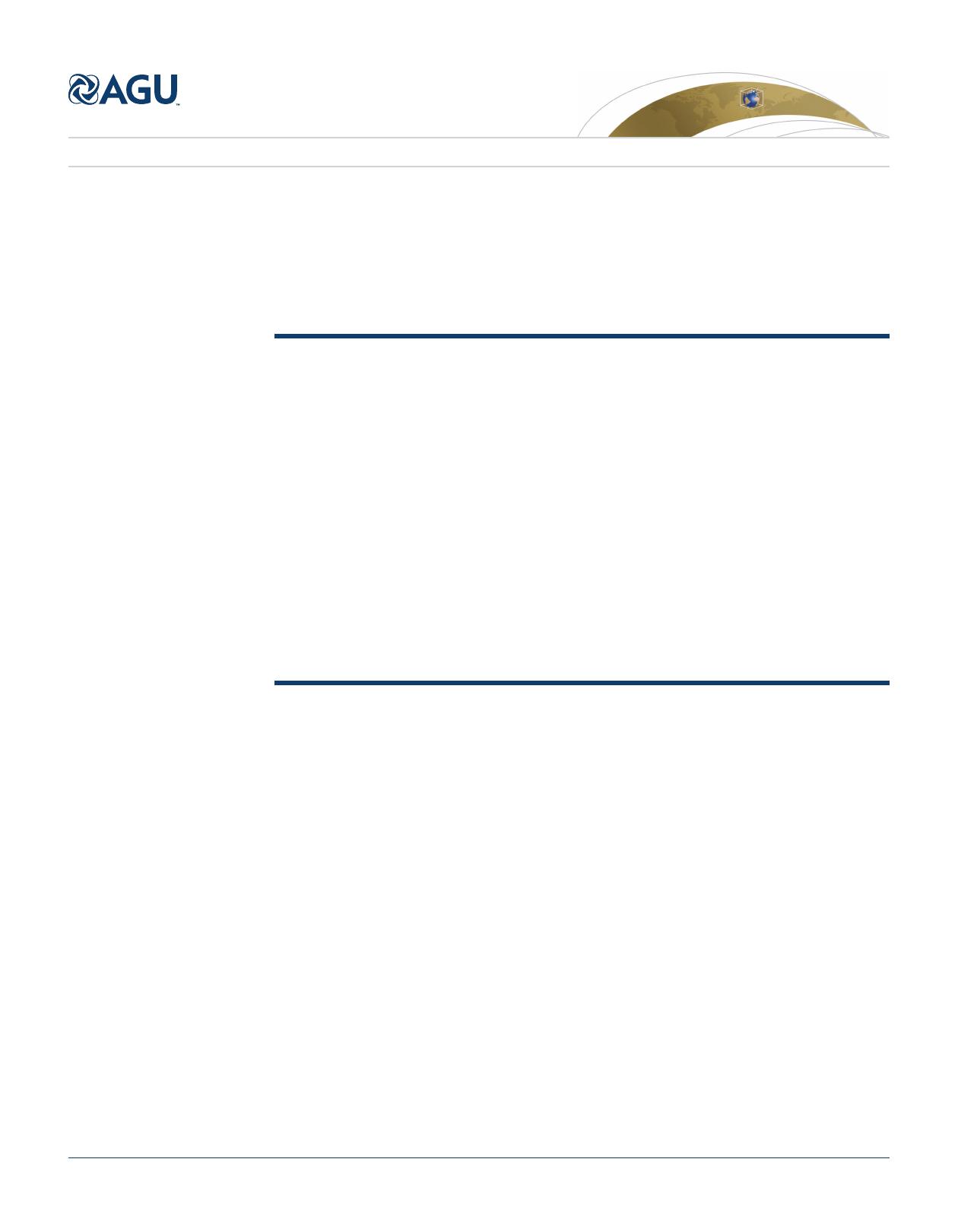
RESEARCH ARTICLE
10.1002/2013GC005148
Serpentinization of mantle-derived peridotites at mid-ocean
ridges: Mesh texture development in the context of tectonic
exhumation
St
ephane Roum
ejon
1
and Mathilde Cannat
1
1
Equipe de G
eosciences Marines, Institut de Physique du Globe de Paris, Sorbonne Paris Cit
e, UMR7154 CNRS, Paris, France
Abstract At slow spreading ridges, axial detachment faults exhume mantle-derived peridotites and
hydrothermal alteration causes serpentinization in a domain extending more than 1 km next to the fault. At
the microscopic scale, serpentinization progresses from a microfracture network toward the center of oli-
vine relicts and forms a mesh texture. We present a petrographic study (SEM, EBSD, and Raman) of the ser-
pentine mesh texture in a set of 278 abyssal serpentinized peridotites from the Mid-Atlantic and Southwest
Indian Ridges. We show that serpentinization initiated along two intersecting sets of microfractures that
have consistent orientations at the sample scale, and in at least one studied location, at the 100 m scale. We
propose that these microfractures formed in fresh peridotites due to combined thermal and tectonic
stresses and subsequently served as channels for serpentinizing fluids. Additional reaction-induced cracks
developed for serpentinization extents <20%. The resulting microfracture network has a typical spacing of
60 mm but most serpentinization occurs next to a subset of these microfractures that define mesh cells
100–400 mm in size. Apparent mesh rim thickness is on average 33 619 mm corresponding to serpentiniza-
tion extents of 70–80%. Published laboratory experiments suggest that mesh rims formation could be com-
pleted in a few years (i.e., quasi instantaneous at the plate tectonic timescale). The depth and extent of the
serpentinization domain in the detachment fault’s footwall are probably variable in time and space and as a
result we expect that the serpentine mesh texture at slow spreading ridges forms at variable rates with a
spatially heterogeneous distribution.
1. Introduction
The oceanic lithosphere formed at slow (<4 cm/yr) [e.g., Small, 1998] spreading ridges contains a variable
amount of serpentinized peridotite in the first kilometers. These rocks, associated with gabbroic bodies,
have been widely observed and sampled along the Mid-Atlantic Ridge [Karson et al., 1987; Cannat et al.,
1992, 1995b, 1997; Kelemen et al., 2004; Gr
acia et al., 2000], the Gakkel ridge [Michael et al., 2003], and the
Southwest Indian Ridge [Dick, 1989; Dick et al., 2003; Seyler et al., 2003; Sauter et al., 2013]. They are exhumed
along large offset normal faults, also called detachment faults [Karson, 1990; Cannat et al., 1992, Cann et al.,
1997; Lavier et al., 1999; Smith et al., 2006]. Microseismicity distribution [Toomey et al., 1985; deMartin et al.,
2007] reveals that the detachment faults extend down to at least 8 km on axis and geological observations
show that the emergence angle of these faults at the seafloor is locally <10[Smith et al., 2006]. Together,
these results suggest that axial detachments have a concave-downward shape, corresponding to a flexure
of the footwall as it is exhumed (Figure 1). Paleomagnetic data acquired on drilled samples are also consist-
ent with rotations of 46–80in the footwall of Mid-Atlantic Ridge detachments [Garc
es and Gee, 2007; Mor-
ris et al., 2009; MacLeod et al., 2011]. Axial detachment faults can therefore be sketched as conveyor belts for
the exhumation of mantle-derived peridotites (Figure 1): these rocks are progressively uplifted from the
base of the brittle lithosphere toward shallower levels in which hydrothermal circulation and serpentiniza-
tion are active.
Serpentinization has significant geodynamic and environmental consequences. It modifies the rheology of
the oceanic lithosphere [Reinen et al., 1994; Escart
ın et al., 1997] and may help localizing the deformation
along fault planes. Serpentinization also consumes large volumes of water and produces hydrogen [Frost,
1985; Charlou et al., 2002], part of which combines with dissolved CO
2
[Berndt et al., 1996] to produce meth-
ane anomalies in the water column [Charlou and Donval, 1993; Charlou et al., 1998]. These hydrogen and
methane fluxes provide energy to sustain microbial systems within the substratum and at hydrothermal
Key Points:
Serpentinization occurs along
thermal, tectonic, and reaction-
induced fractures
Serpentinization may occur quasi
instantly at exhumation time scales
The depth and extent of
serpentinization are variable in space
and time
Supporting Information:
ReadMe
Table S1_v3
text_Auxiliary_material
Fig_annexe_opx_reviews
Fig_annexe_EBSDmaps
Correspondence to:
S. Roum
ejon,
Citation:
Roum
ejon, S., and M. Cannat (2014),
Serpentinization of mantle-derived
peridotites at mid-ocean ridges: Mesh
texture development in the context of
tectonic exhumation, Geochem.
Geophys. Geosyst.,15, 2354–2379,
doi:10.1002/2013GC005148.
Received 13 NOV 2013
Accepted 19 MAY 2014
Accepted article online 23 MAY 2014
Published online 10 JUN 2014
ROUM
EJON AND CANNAT V
C2014. American Geophysical Union. All Rights Reserved. 2354
Geochemistry, Geophysics, Geosystems
PUBLICATIONS