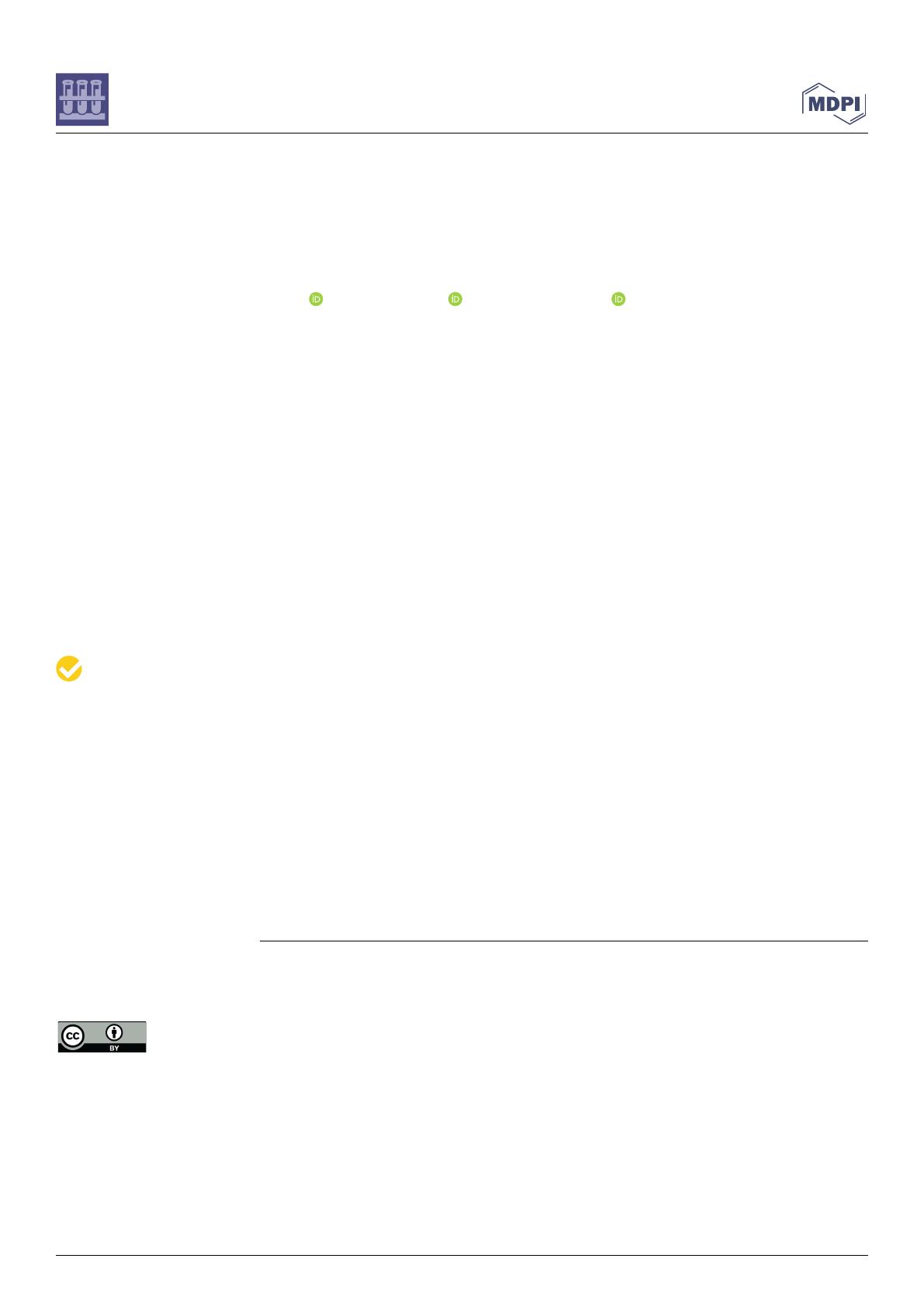
Article
Power Quality Analysis of the Output Voltage of AC Voltage
and Frequency Controllers Realized with Various Voltage
Control Techniques
Naveed Ashraf 1, Ghulam Abbas 1,* , Rabeh Abbassi 2and Houssem Jerbi 3
Citation: Ashraf, N.; Abbas, G.;
Abbassi, R.; Jerbi, H. Power Quality
Analysis of the Output Voltage of AC
Voltage and Frequency Controllers
Realized with Various Voltage
Control Techniques. Appl. Sci. 2021,
11, 538. https://doi.org/
10.3390/app11020538
Received: 27 November 2020
Accepted: 5 January 2021
Published: 7 January 2021
Publisher’s Note: MDPI stays neu-
tral with regard to jurisdictional clai-
ms in published maps and institutio-
nal affiliations.
Copyright: © 2021 by the authors. Li-
censee MDPI, Basel, Switzerland.
This article is an open access article
distributed under the terms and con-
ditions of the Creative Commons At-
tribution (CC BY) license (https://
creativecommons.org/licenses/by/
4.0/).
1Department of Electrical Engineering, The University of Lahore, Lahore 54000, Pakistan;
2Department of Electrical Engineering, College of Engineering, University of Ha’il, Hail 1234, Saudi Arabia;
3Department of Industrial Engineering, College of Engineering, University of Ha’il, Hail 1234, Saudi Arabia;
Abstract:
Single-phase and three-phase AC-AC converters are employed in variable speed drive,
induction heating systems, and grid voltage compensation. They are direct frequency and voltage
controllers having no intermediate power conversion stage. The frequency controllers govern the
output frequency (low or high) in discrete steps as per the requirements. The voltage controllers
only regulate the RMS value of the output voltage. The output voltage regulation is achieved on the
basis of the various voltage control techniques such as phase-angle, on-off cycle, and pulse-width
modulation (PWM) control. The power quality of the output voltage is directly linked with its
control techniques. Voltage controllers implemented with a simple control technique have large
harmonics in their output voltage. Different control techniques have various harmonics profiles
in the spectrum of the output voltage. Traditionally, the evaluation of power quality concerns is
based on the simulation platform. The validity of the simulated values depends on the selection of
the period of a waveform. Any deficiency in the selection of the period leads to incorrect results.
A mathematical analytical approach can tackle this issue. This becomes important to analytically
analyze the harmonious contents generated by various switching control algorithms for the output
voltage so that these results can be successfully used for power quality analysis and filtering of
harmonics components through various harmonics suppression techniques. Therefore, this research
is focused on the analytical computation of the harmonics coefficients in the output voltage realized
through the various voltage and frequency control techniques. The mathematically computed results
are validated with the simulation and experimental results.
Keywords:
voltage and frequency controller; grid voltage compensation; power quality; PWM
control; harmonics coefficients
1. Introduction
1.1. Problem Statement
Power quality is one of the major concerns in today’s modern power system. In tradi-
tional generation and distribution systems, the issue of low power quality is meaningless as
the connected load is linear such as incandescent lamps and heating load. The speed of the
rotating loads is governed via their voltage control that is achieved through conventional
approaches. That includes the use of auto-transformers, transformer tap-changing mecha-
nisms, and variable resistance. These power control mechanisms are inefficient and are
replaced with switching converters nowadays. The power electronic converters are plying
a vital role in the development of modern-day life by converting one form of electric power
into another form. The converted output in the power conversion process is not always
Appl. Sci. 2021,11, 538. https://doi.org/10.3390/app11020538 https://www.mdpi.com/journal/applsci