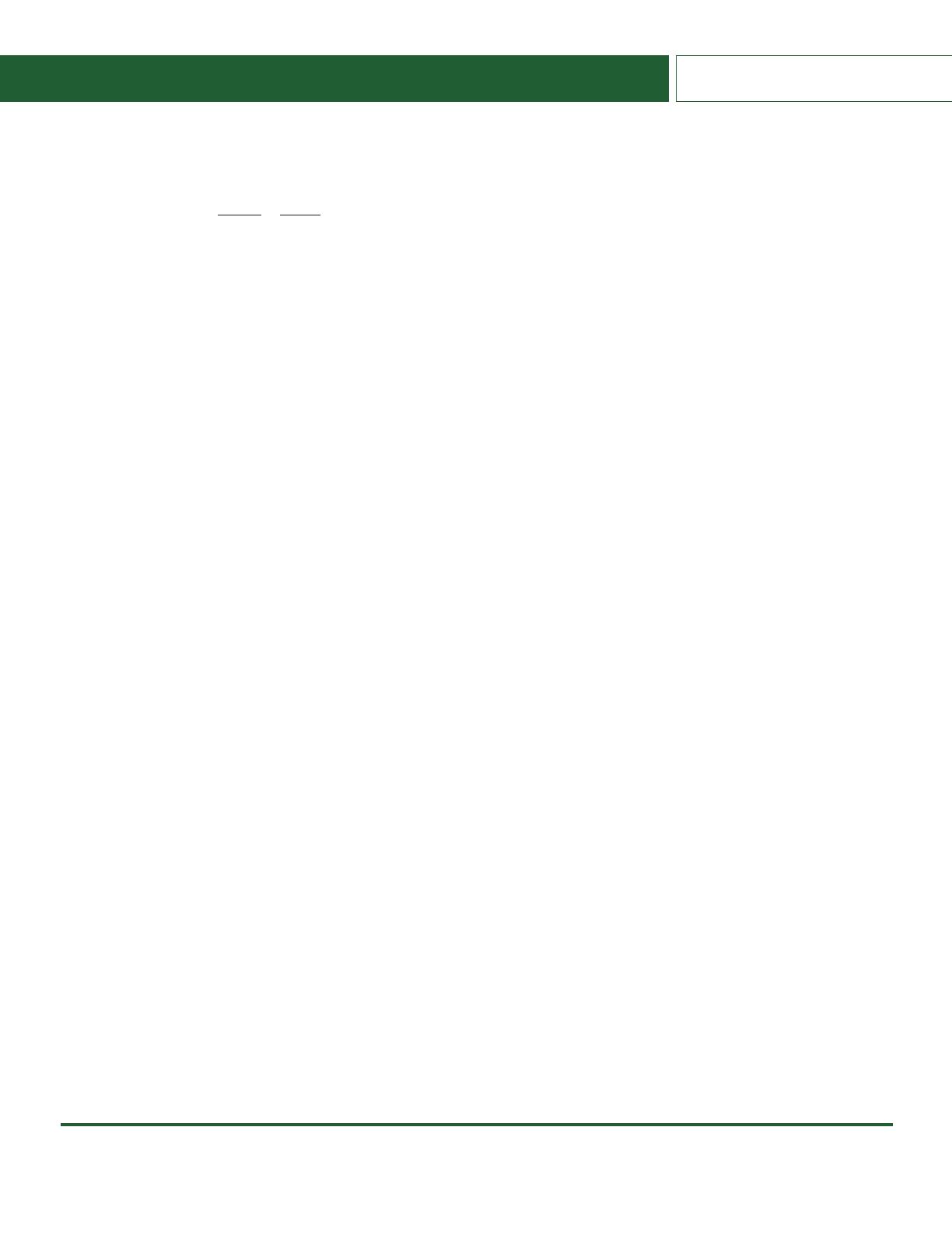
withinthedevicecouldbemadetoapproachthemagneticpressure,
that is if
b¼Pplasma
Pmagnetic ¼nkBT
B2=2l0!1;(1)
then the diamagnetic circulation of electrons about magnetic field lines
would expel the applied field from the core. This “high beta” mode,
sometimes referred to as the “Wiffle ball” after the popular American
children’s toy, was expected to exhibit the favorable particle trapping
properties first described by Grad and Berkowitz
43,44
leading to a sharp
increase in device performance once the high beta mode was obtained.
A number of low beta polywell devices have also been operated
at The University of Sydney (USYD).
45–51
The work has been princi-
pally concerned with the phenomenon of potential well formation and
characterization of the electron trapping properties of the polywell
field configuration. The first USYD polywell, constructed by Carr,
45
consisted of teflon reels fastened together by aluminum brackets.
Electrons were injected using a hollow cathode style electron gun
52
and floating Langmuir probe measurements showed 100–200 V
potential wells over a broad range of background gas pressures. Carr
subsequently upgraded his machine using toroidal aluminum coil
cases that were conformal to the magnetic field. The resulting machine
operated at lower voltages than its predecessor (100–200 V) but pro-
duced potential wells of comparable relative depth (10–20 V). Carr
also used biased Langmuir probe measurements to characterize the
energies of the trapped electrons and proposed a novel energy distri-
bution function.
47
Cornish
49,50
conducted a survey of scaling laws
within the Polywell, with particular consideration given to the depen-
dence of virtual cathode formation on the size and spacing of the mag-
netic field coils. Finally, studies conducted by Ren and Poznic
51
examined electron density and energy distributions in a larger device
using both biased Langmuir probe and radio frequency plasma wave
techniques.
In this paper, we detail the operation of magnetically confined
virtual cathode (MCVC-0), the University of Sydney’s most recent
polywell-style machine. MCVC-0 represents both the largest and high-
est power virtual cathode fusion experiment at the University of
Sydney and was designed to operate in a parameter regime (in terms
of applied voltages and plasma currents) where measurable deuter-
ium–deuterium fusion was possible. Due to size limitations, this
machine consists of two magnetic field coils, rather than the traditional
six, arranged such that they form a biconic cusp. As such, the device
maynolongerbereferredtoasapolywell, and hence we adopt the
more generalized nomenclature magnetically confined virtual cathode.
In Sec. II, we describe the MCVC-0 system and the associated diagnos-
tics. In Sec. III, we present results from single-ended floating probe
measurements of the MCVC-0 plasma and illustrate significant dis-
crepancies between the measured electrostatic profiles and the
expected potential wells. In Sec. IV, we outline a theoretical model
based on the solution of the Poisson equation in the presence of a con-
ductive medium and demonstrate how anisotropic plasma conductiv-
ity sufficiently accounts for the unexpected behavior of the MCVC-0
machine. It is found that the electric potential within the device is
dominated by those electrostatic boundaries that are intersected by
magnetic field lines, which has significant implications for the design
of future MCVC/polywell style fusion machines.
II. APPARATUS
A. MCVC-0 device
The MCVC-0 device was constructed within a cylindrical vacuum
chamber 300 mm in diameter and 300 mm in length. A pair of
100 turn magnetic field coils (152 mm diam, 1770 lHand0:15 X)
were situated within toroidal metal cases, which were the positive elec-
trodes in the MCVC-0 system. The tori were constructed from 316
stainless steel and had major and minor radii of approximately 76 and
30 mm, respectively, and a wall thickness of 4 mm. In order to allow
biasing of the cases relative to the field coils, the coils were supported
freefloatingwithinthetoriusingsetsofinterlockinginsulatingcollars
made from polyether–ether–ketone (PEEK). The coils were mounted
on a support frame consisting of a pair of stainless steel plates fastened
together by three vertical struts. The magnetic field coils were mounted
to the frame on PEEK bushings, such that the cases could be biased
610 kV relative to the support structure. The PEEK supports were
machined with multiple fins to increase the arc creep distance and
were sheathed in 60 mm O.D. alumina ceramic tube to prevent sput-
tering damage. In order to improve the high vacuum compatibility of
the apparatus, all steel components were baked at 500 Cfor72h.The
coil support structure is depicted in Fig. 1. Note that the magnetic field
lines within the device are everywhere conformal to the toroidal cases
such that particle collection on the electrodes occurs transverse to the
magnetic field. This so-called “magnetic shielding” reduces the col-
lected current during a shot, thereby improving the efficiency of the
device.
The magnetic field coils were driven by a 108 mF, 900 V capaci-
tor bank resulting in a maximum coil current of 2000 A. The peak
achievable magnetic field strength, when measured in the center of the
coils, was 1.15 T. The coil cases were biased at a maximum voltage of
6 kV, switched to the cases from a 180 lF capacitor using a MOSFET
switch. Electron beam injection was necessary for the formation of a
virtual cathode. A thermionic electron source was therefore con-
structed in the lower, axial vacuum port such that electrons could be
injected through the face of the lower magnetic field coils. A custom
filament support stage was positioned within the lower port of the vac-
uum chamber and populated with six 12 V, 50 W tungsten filaments
obtained from halogen light bulbs. The filament stage was supported
on ceramic rods such that it could be biased to 2000 V with respect
to the vacuum chamber. A grounded molybdenum mesh spanned the
port opening to accelerate the thermionic electrons into the chamber.
Theentireelectrongunassemblywassheathedwitha62mminside
diameter borosilicate glass tube in order to provide additional electrical
standoff between the floating filament stage and the wall of the vac-
uum port. In all experiments the electron gun current was set to its
maximum value of 150 mA, at an injection energy of 1 kV. The total
energy gained by an electron as it enters the core of the device is there-
fore the applied coil bias plus 1 keV.
Prior to all experiments, the MCVC-0 system was pumped down,
with baking at 165 C, until the ultimate base pressure of
1107Torr was reached. Optical spectra obtained during plasma
shots at base pressure were found to be dominated by strong HaH
Balmer lines, identifying the primary gas component as residual atmo-
spheric water. All experimental runs were therefore conducted without
the addition of hydrogen or deuterium gas and hence all presented
results were obtained using the base system pressure of
1107Torr. During a typical shot, the electron gun was activated
Physics of Plasmas ARTICLE scitation.org/journal/php
Phys. Plasmas 28, 042702 (2021); doi: 10.1063/5.0040792 28, 042702-2
Published under license by AIP Publishing