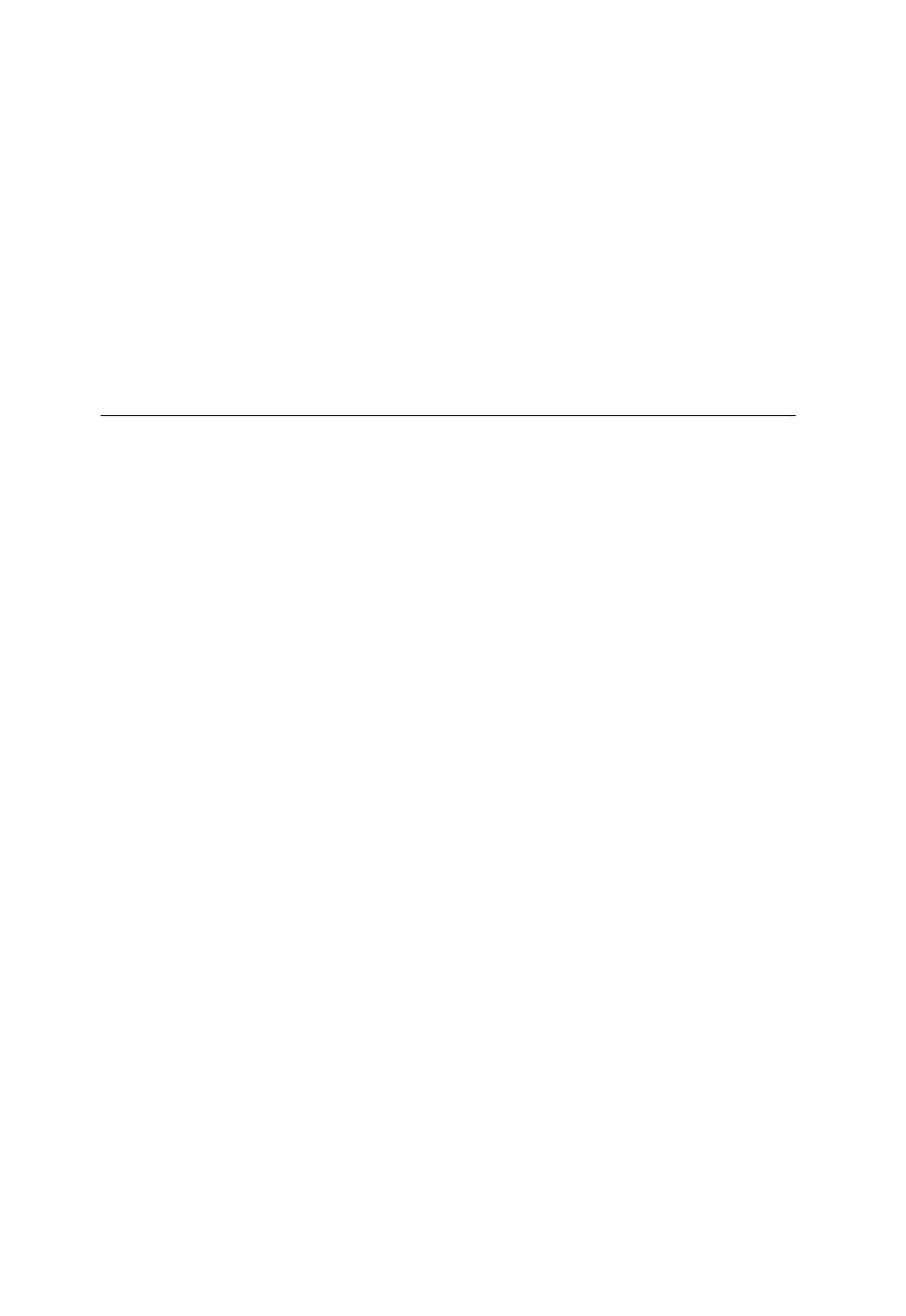
The body segmental analysis and force platform techniques were in agreement at all walking
speeds. Discrepancies between the sacral marker method and the other two techniques were
explained using a simple model; the reciprocal configuration of the legs during double support
phase significantly raises the position of the BCOM within the trunk at longer step lengths,
corresponding to faster walking speeds. The sacral marker method may provide a reasonable
approximation of vertical BCOM motion at slow and freely selected speeds of able-bodied
walking. However, the body segmental analysis or force platform techniques will probably
yield better estimates at faster walking speeds or in persons with gait pathologies.
2003 Elsevier B.V. All rights reserved.
PsycINFO classification: 2260
Keywords: Human movement; Gait analysis; Center of mass
1. Introduction
Translation of the body center of mass (BCOM) from one place to another is a
fundamental objective of walking. Normal human walking is characterized by a peri-
odic vertical displacement of the BCOM that moves through a complete cycle of ver-
tical motion with each step, or two cycles during each stride. The peak-to-peak
amplitude of the vertical BCOM displacement, referred to as the vertical excursion,
is generally regarded to be about 4–5 cm for adult ambulators at their freely selected
walking speed (Murray, Drought, & Kory, 1964; Inman, Ralston, & Todd, 1994;
Saunders, Inman, & Eberhart, 1953). Investigators have used vertical BCOM motion
during walking to estimate mechanical energy changes (Cavagna, 1975; Iida &
Yamamuro, 1987; Tesio, Civaschi, & Tessari, 1985; Tesio, Lanzi, & Detrembleur,
1998a, 1998b), to gauge efficiency (Cavagna, Tesio, Fuchimoto, & Heglund, 1983;
Saunders et al., 1953), to estimate work (Cavagna, Saibene, & Margaria, 1963; Cav-
agna & Margaria, 1966; Donelan, Kram, & Kuo, 2002), to describe symmetry (Cav-
agna et al., 1983; Crowe, Schiereck, & Keessen, 1992; Gard, Knox, & Childress,
1996), and as an indicator of the overall quality of gait (Bowker & Hall, 1975; Saun-
ders et al., 1953). All of these applications rely on accurate determination of vertical
BCOM motion.
Investigators have developed a number of basic methodologies for calculating
BCOM motion during walking. Some of the methods utilize kinematic data acquired
from markers that are placed on the body, and others utilize kinetic data acquired
from force platforms. One of the simplest kinematic methods, the sacral marker
method, uses a single marker placed on the sacrum to approximate BCOM motion.
A more sophisticated approach, the segmental analysis method, uses multiple mark-
ers to measure body segment positions, and incorporates an anthropometric model
to calculate segmental center of mass positions. These segmental center of mass posi-
tions are then used to calculate the BCOM. In contrast, the force platform method
uses measured ground reaction forces to calculate BCOM motion based upon New-
ton’s Second Law, which states that the net external force acting upon a body is
598 S.A. Gard et al. / Human Movement Science 22 (2004) 597–610