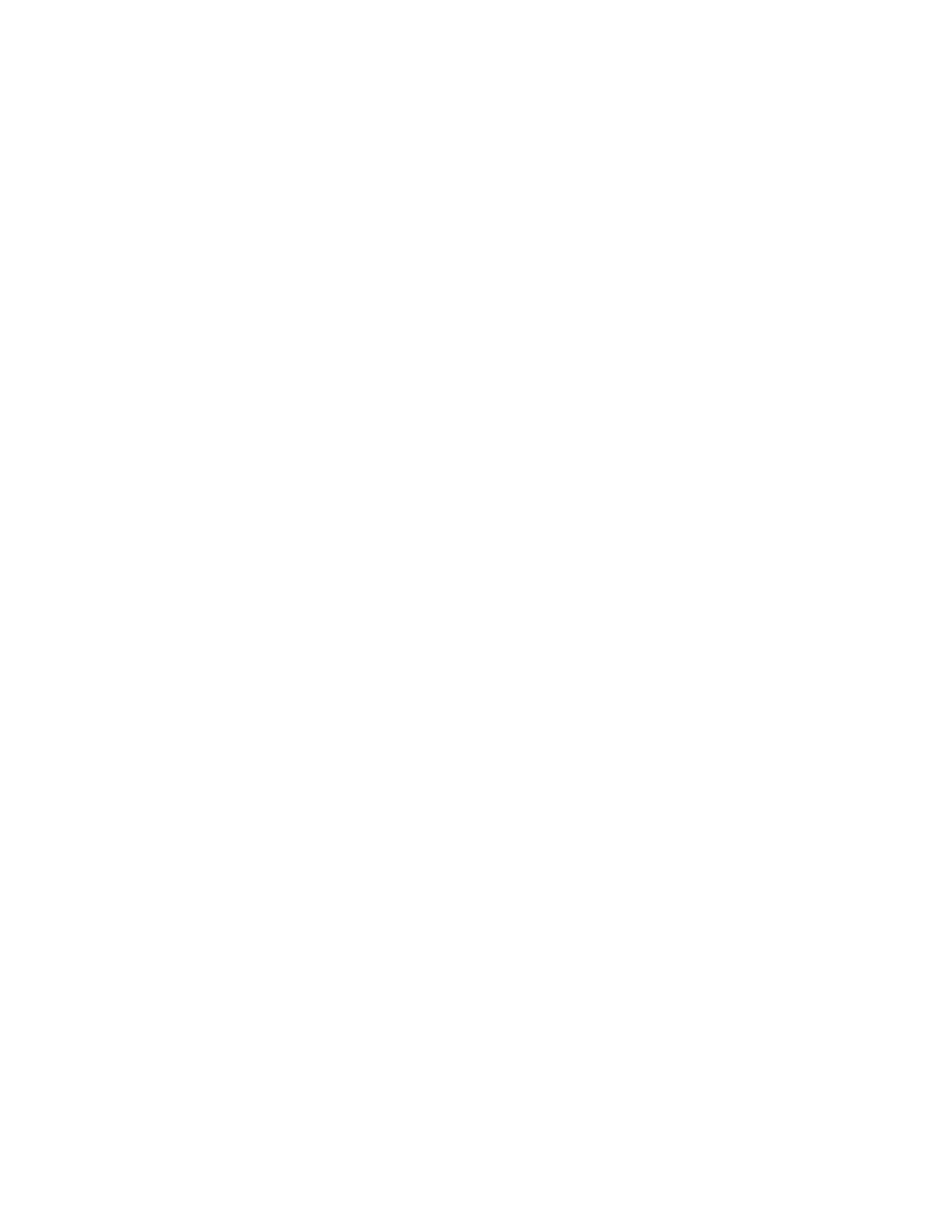
Acknowledgements
I would like to thank my supervisor Dr. Robin Yates for his guidance and support for this
study. Working under the supervision of a scientist as enthusiastic and motivated as him, has
been a great experience. Not only was his door always open for discussions, but he was also
always enthusiastic to troubleshoot, teach or work at the bench together.
I would like to thank Dr. Yan Shi, Dr Faizal Careem and Dr. Derek Mckay for investing
their time to be on my supervisory committee.
I would like to thank Dr. Neil McKenna for helping me carry out some of the ex vivo
experiments, proof-reading my thesis, and for all his invaluable suggestions for troubleshooting
experiments. He is a great co-worker who always greatly contributes to making working in lab
super fun for all lab members. I would also like to thank him for his patience when I was slow to
learn, and for having faith in my abilities.
I would like to thank everyone in the Yates lab - Dale Balce for his invaluable suggestions
on phagosomal assays, Jason Lemmon for valuable tips on improving writing skills. I would like
to thank Euan Allan, Jason Abboud, Pankaj Tailor, Paymon, Bernard Euanchak, Raymond Lam,
Samuel Lee for being great people to work with.
I would like to thank our collaborator Dr. John Hardings for sending us exvivo samples.
Finally, I would like to thank my family and friends for their moral support, particularly
my parents for having faith in my abilities and my sister who made me see “the other side of
the coin”. Moving half way across the globe can be challenging. I would like to thank all who
made this transition easy – particularly Meenakshi Rawat, Demyana Boles and my flatmate
Liane Babes.