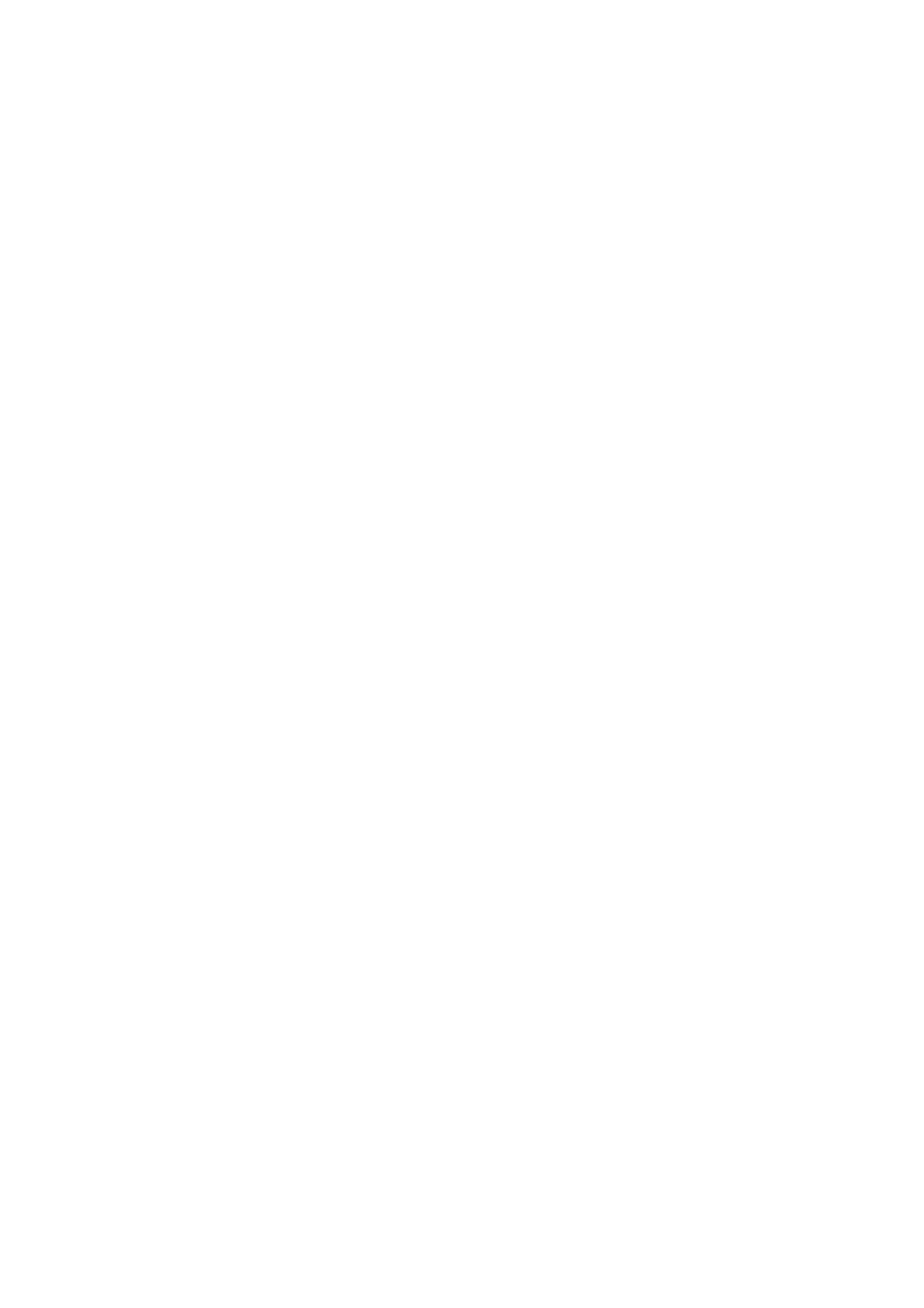
LaDra.NATÀLIAMAJÓiMASFERRER,professoratitulardelDepartamentdeSanitati
d’AnatomiaAnimalsdelaFacultatdeVeterinàriadelaUniversitatAutònomadeBarcelonai
investigadoraadscritaalCentredeRecercaenSanitatAnimal(CReSA),ilaDra.ROSERDOLZ
iPASCUAL,investigadoradelCReSA,
Certifiquen:
Quelamemòriatitulada“AvianInfluenzaInfectionDynamicsinMinorAvianSpecies”
presentadapernaKateriBertraniDolsperal’obtenciódelgraudeDoctor,s’harealitzat
sotalasevadireccióisupervisiói,considerant‐laacabada,n’autoritzenlasevapresentació
pertaldeseravaluadaperlacomissiócorresponent.
Ipertalqueconstialsefectesoportuns,signenelpresentcertificataBellaterra,a19de
marçde2013.
Dra.NatàliaMajóiMasferrerDra.RoserDolziPascualKateriBertraniDols
DirectoraDirectoraDoctoranda