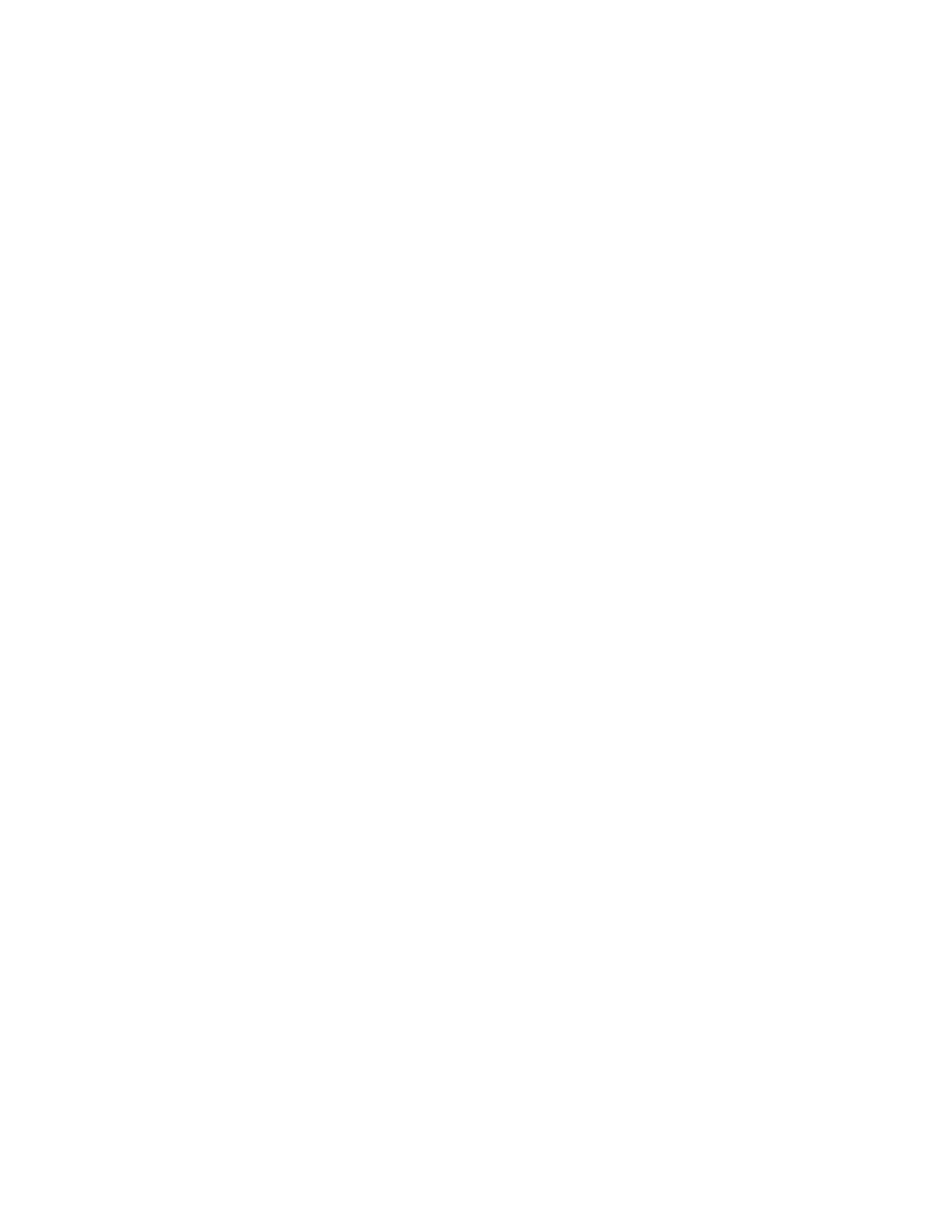
iv
ACKNOWLEDGEMENTS
First of all, I would like to acknowledge the University of Calgary, Faculty of Veterinary
Medicine for providing me the opportunity to conduct my MSc studies. The majority of this
work was kindly funded by the Alberta Livestock and Meat Agency (ALMA) and the Canadian
Poultry Research Council (CPRC).
I am forever grateful to my supervisor, Dr. Faizal Careem, and my co-supervisor, Dr. Markus
Czub, for not just giving me the opportunity to work in their laboratories, but also for inspiring
me to think critically and giving me constructive advice on the delivery of effective oral and
written presentations. The feedbacks that were given throughout my studentship are invaluable
and will be useful in my future professional career.
I thank the members of my graduate research committee, Dr. Hermann Schaetzl and Dr.
Karen Liljebjelke, for providing advice and direction, which have made the completion of this
master’s thesis possible. In particular, I thank Dr. Frank van der Meer for supporting me
intellectually and academically to become more successful during my MSc graduate program.
Also, I thank other members of Careem lab, i.e. Stephan Bonfield, Dae-Sun Kim, Amber
Kameka, Jasmine Hui for any technical and infrastructural support. Much appreciation also goes
to Dr. Rkia Dardari for guiding me in cell culture techniques, Dr. Constance Finney for the
guidance on flow cytometry techniques, Dr. Robin Yates (from University of Calgary) and Dr.
Shayan Sharif (from University of Guelph) for providing me with macrophage cell lines, flow
cytometry core facility at University of Calgary for running flow cytometry samples, and
Southern Alberta Cancer Research Institute for kindly allowing me to use micro plate reader for
reading my NO assay samples, and other members of Czub, Van der Meer, Van Marle and