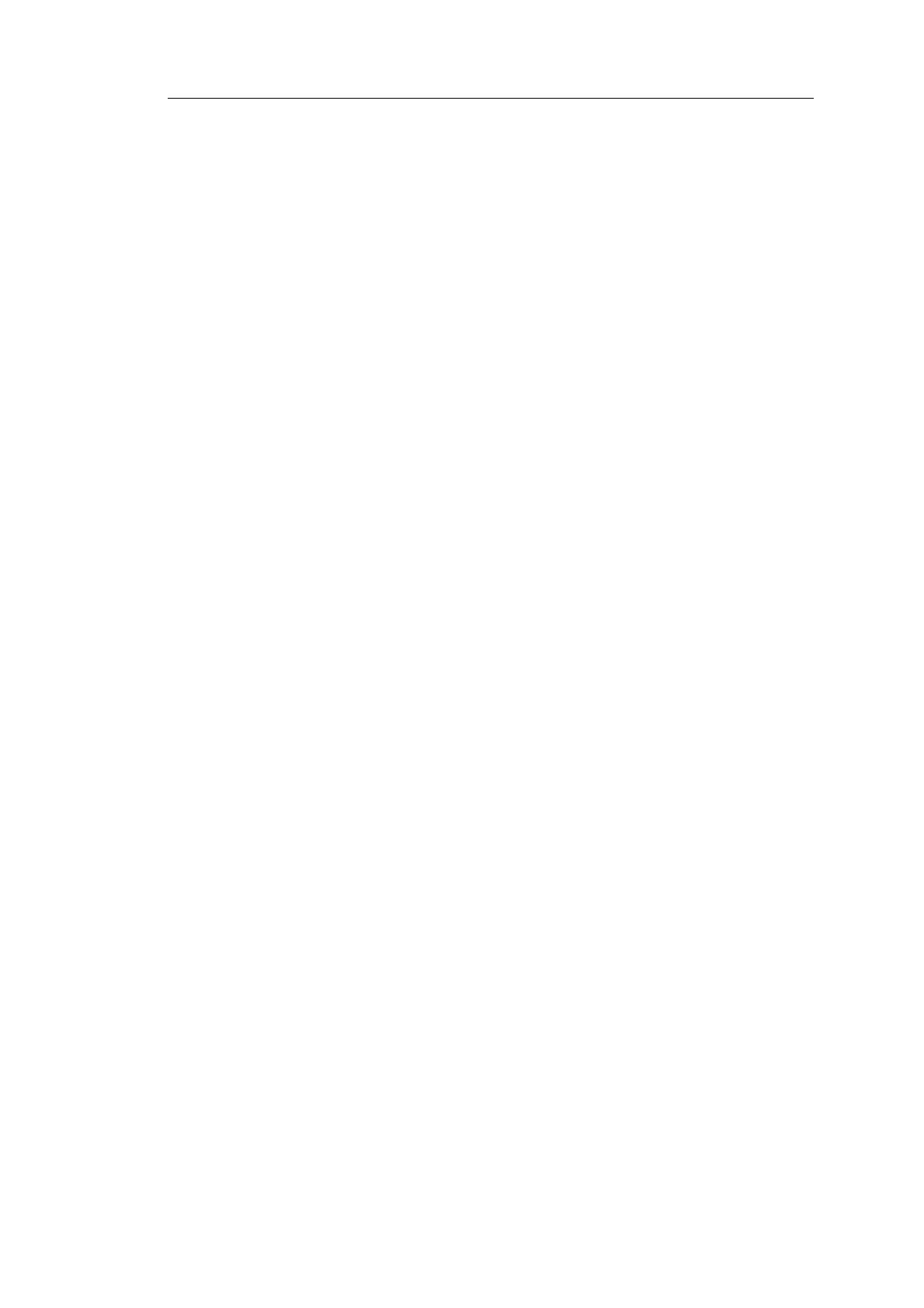
Contents iv
3.2.6.4 Spectral fitting . . . . . . . . . . . . . . . . . . . . . . . . 32
3.2.6.5 2.5 years of contemporaneous Radio and GeV data . . . . 32
3.2.6.6 Summary . . . . . . . . . . . . . . . . . . . . . . . . . . . 32
3.3 LS I +61◦303 Longterm Gamma-Ray Variability . . . . . . . . . . . . . . . 33
3.3.1 Data and Analysis settings . . . . . . . . . . . . . . . . . . . . . . 34
3.3.2 Results . . . . . . . . . . . . . . . . . . . . . . . . . . . . . . . . . 35
3.3.3 Interpretation . . . . . . . . . . . . . . . . . . . . . . . . . . . . . . 40
3.3.4 Multi-wavelength context . . . . . . . . . . . . . . . . . . . . . . . 42
3.3.4.1 X-rays . . . . . . . . . . . . . . . . . . . . . . . . . . . . . 42
3.4 Pulsed fraction for high mass X-ray binaries . . . . . . . . . . . . . . . . . 46
3.4.1 Comparison of the limits with X-ray pulsed fractions of known
pulsars.................................. 47
4 PART II: Magnetars 54
4.1 Overview and motivation . . . . . . . . . . . . . . . . . . . . . . . . . . . 54
4.2 Magnetars observations at TeV with the MAGIC telescopes . . . . . . . . 56
4.2.1 The observed magnetars . . . . . . . . . . . . . . . . . . . . . . . . 56
4.2.2 Analysis and Data . . . . . . . . . . . . . . . . . . . . . . . . . . . 57
4.2.3 Results . . . . . . . . . . . . . . . . . . . . . . . . . . . . . . . . . 58
4.3 Magnetars observations at GeV with the Fermi Large Area Telescope . . 61
4.3.1 Observation and data reduction . . . . . . . . . . . . . . . . . . . . 61
4.3.2 Likelihood analysis and results . . . . . . . . . . . . . . . . . . . . 62
4.3.3 Sources with high TS values . . . . . . . . . . . . . . . . . . . . . . 63
4.3.4 Upper limits evaluation . . . . . . . . . . . . . . . . . . . . . . . . 65
4.3.5 Timing analysis . . . . . . . . . . . . . . . . . . . . . . . . . . . . . 65
4.3.6 Results and Discussion . . . . . . . . . . . . . . . . . . . . . . . . . 66
5 PART III: Prospects 68
5.1 Description of CTA . . . . . . . . . . . . . . . . . . . . . . . . . . . . . . . 68
5.2 Motivation . . . . . . . . . . . . . . . . . . . . . . . . . . . . . . . . . . . 71
5.2.1 CTA flux error reduction in known TeV sources . . . . . . . . . . . 73
5.2.2 Short timescale flux variability . . . . . . . . . . . . . . . . . . . . 76
5.2.3 Sensitivity to spectral shape variations . . . . . . . . . . . . . . . . 78
5.2.4 Exploring the colliding winds of massive star binary systems . . . 79
5.3 Summary and conclusion . . . . . . . . . . . . . . . . . . . . . . . . . . . 80
Bibliography 81