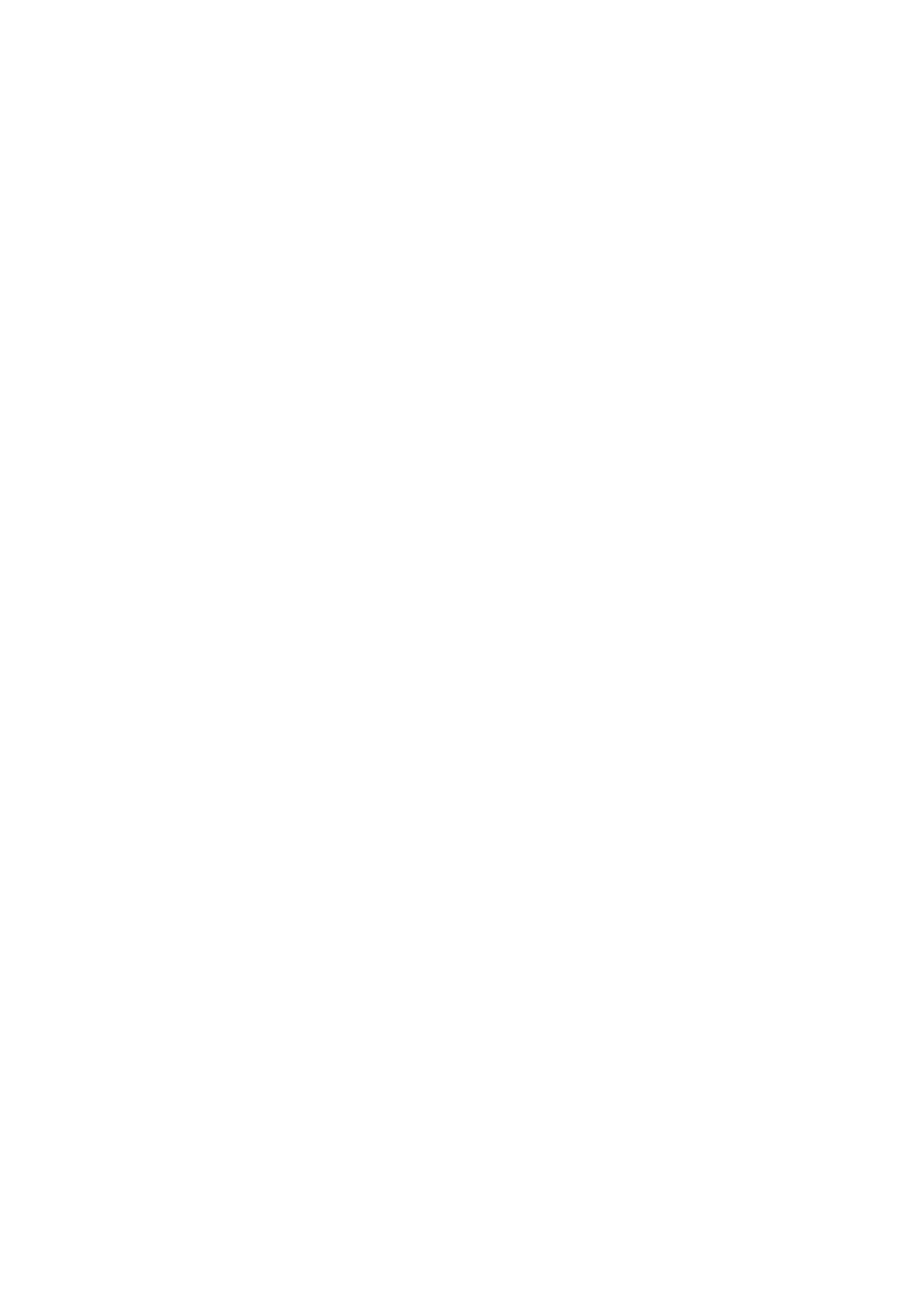
Enric M. Mateu de Antonio, professor titular del Departament de Sanitat i d’Anatomia
Animals de la Facultat de Veterinària i investigador adscrit a l’IRTA
Declara
Que la memòria titulada: “Transmission of Porcine reproductive and respiratory
syndrome virus (PRRSV): assessment of the basic reproduction rate in different
conditions”, presentada per l’Emanuela Pileri per a l’obtenció del grau de Doctor en
Veterinària, s’ha realitzat sota la meva direcció en el programa de doctorat de
Medicina i Sanitat Animals, del Departament de Sanitat i d’Anatomia Animals, opció
Sanitat Animal.
I per a que consti als efectes oportuns, signo la present declaració en Bellaterra, 16 de
Setembre de 2015:
Dr. Enric M. Mateu Emanuela Pileri
Director Doctoranda