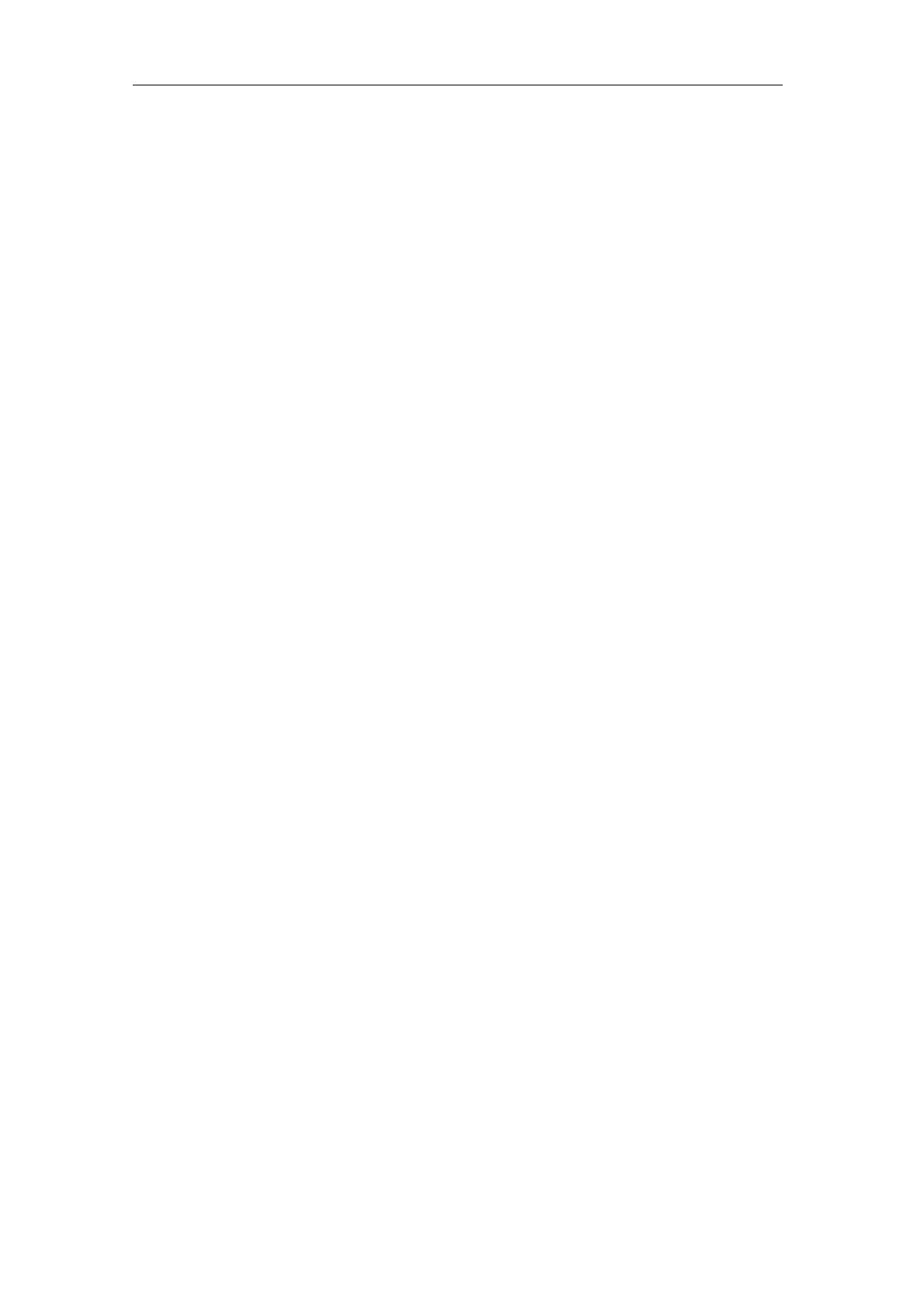
Abbreviations
GK glucokinase
GLP-1 glucagon-like peptide 1
h hour
hCMV human cytomegalovirus
HC-Ad High capacity adenoviral vectors
HD-Ad Helper-dependent adenoviral vectors
HEK Human Embryo Kidney
HIV human immunodeficiency virus
HLA Human leukocyte antigen
HPRT Hypoxanthine-guanine phosphoribosyltransferase
IFN-beta interferon
IFN/IGF-I double transgenic mice RIP/IFN and RIP/IGF-I
IGFBP Insulin-like growth factor binding protein
IGF-I Insulin-like growth factor-I
IGF-II Insulin-like growth factor-II
IGF-IR Insulin-like growth factor receptor-I
IL interleukine
IM Intramuscular
iNOS inducible nitric oxide sinthase
Ip intraperitoneal
IP3 inositol triphosfphate
IR Insulin receptor
IRS-1 Insulin receptor substrate 1
IRS-2 Insulin receptor substrate 2
IU infection units of adenoviral particles
Kb Kilobase
KDa kiloDalton
KO knock-out
M molar (mol/l)
MAPK mitogen-activated protein kinase
MCS Multiple Cloning Site
mg milligrams
MHC major histocompatibility complex
min minute/s
ml millilitre
MLD multiple low dosis
mM millimolar
mRNA RNA messenger
NO Nitric oxide
NOD non obese diabetic
o/n over night
OD optical density
32P Phosphor 32
PBS Phosphate-Buffered Saline
PCR Polymerase Chain Reaction
PDX-1 pancreatic duodenal homeobox gene