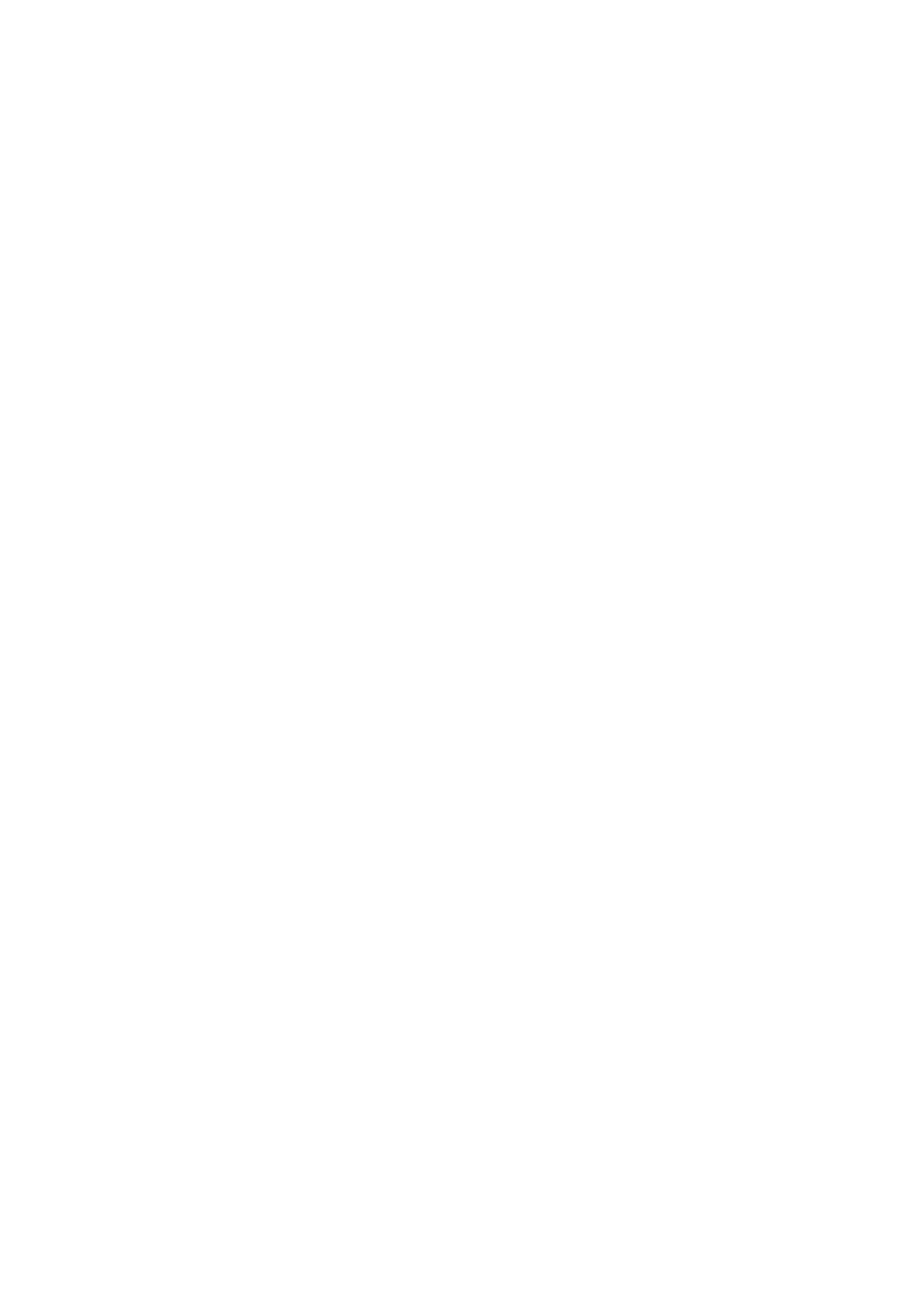
thesis suggests the absence of obvious role of domestic pigs in SBV life-cycle. The absence of
RNAemia is indeed a strong indication that further spread of SBV from the pigs to the
Culicoides during a blood meal of the vector is not likely to occur, therefore making
impossible an SBV transmission. The limited and temporary seroconversion observed after
SBV inoculation in only half of the inoculated piglets and the absence of seroconversion
reported in a limited number of field collected samples support this consideration.
To prevent SBV progression, it was crucial to further study the pathogenesis of SBV.
The Study 1 proved that the most important clinical impact of SBV was the consequence of
the malformed new-born; hereto it was particularly crucial to improve the knowledge on the
development of the SBV-related teratogenic effects. In this respect, experimental infection of
pregnant sheep with SBV constituted an appropriate research approach. An experimental
model was therefore essential to standardize. This thesis contributed to the standardization of
in vivo experiments (in collaboration with another working group) by determining the
minimum infectious dose of an SBV infectious inoculum. This reference infectious serum
must contain approximately 20 TCID50 to induce a homogeneous effective infection in sheep.
This dose is rather low and could be inoculated by a single Culicoides under natural
conditions. Beyond this minimum infectious dose, no dose dependent effect was observed in
productively inoculated ewes, either in the duration of the RNAemia, the quantity of SBV
RNA detected by rRT–PCR in the blood, or in the number of SBV RNA copies present in the
organs collected at necropsy.
The experimental model developed (partly) in the Study 3 was used to inoculate
pregnant ewes at day 45 and 60 of gestation, and increase the knowledge on SBV
transplacental transmission. The inoculation induced the persistence of SBV RNA in placental
organs until birth. Schmallenberg virus RNA was recovered from the organs collected at birth
from the lambs of both groups. However, the chance to obtain SBV RNA positive placental