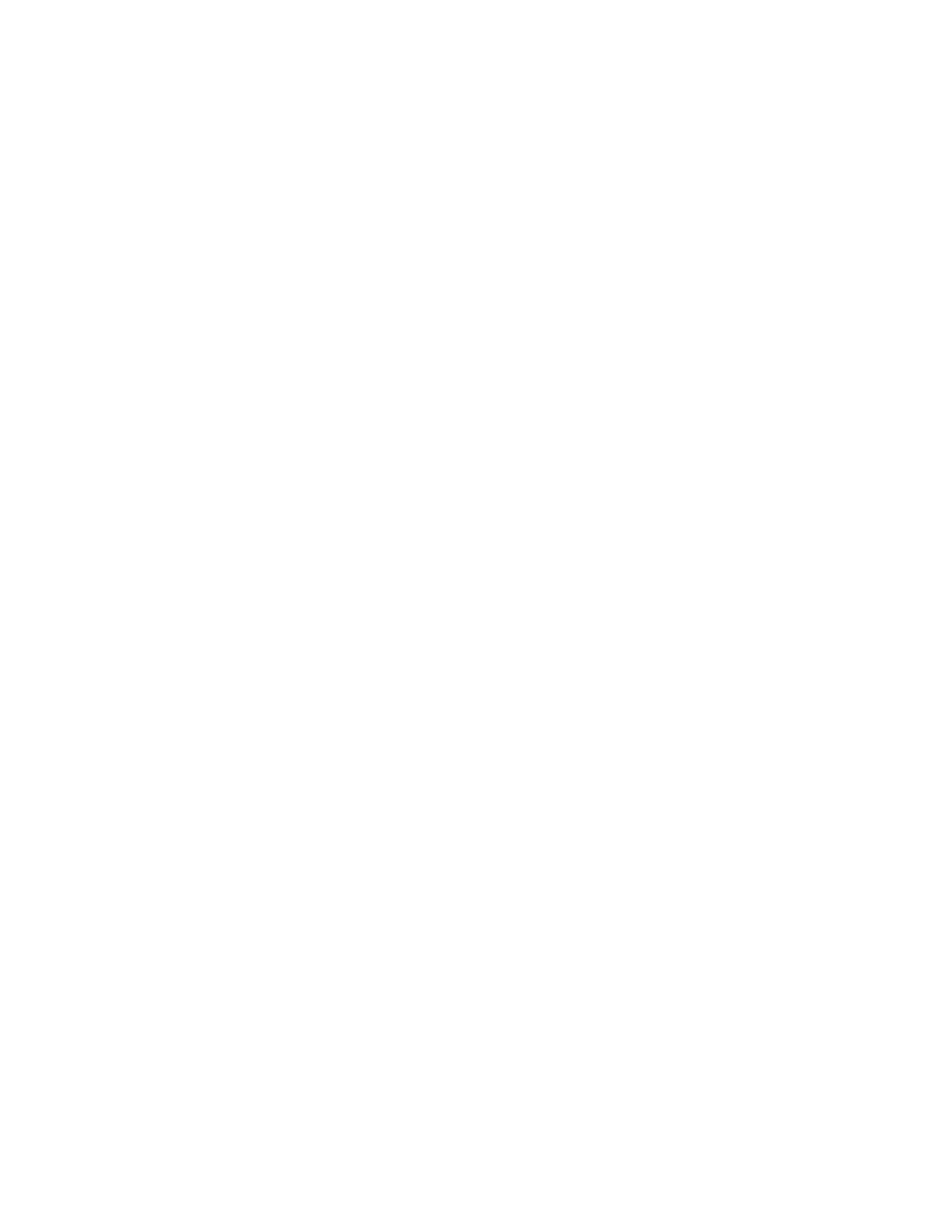
v
REMERCIEMENTS
Je tiens tout d’abord à remercier le Dre Veronika von Messling, ma directrice de
recherche, de m’avoir permis d’entreprendre cette thèse dans son laboratoire et surtout de m’avoir
permis de l’achever. Sans son aide constante, son expérience, sa patience et son dévouement, je
n’aurais pu venir à bout de ce projet. Je tiens à lui exprimer toute ma gratitude.
Je tiens également à remercier mes nombreux collègues de travail avec qui nous avons pu
partager les bons moments propres à la recherche mais qui m’ont aussi permis de garder le moral
lors des coups durs. Je remercie tout particulièrement Danielle pour sa sagesse, Christophe et
Sébastien pour leurs humours, Xiao pour sa candeur et Isabelle pour ses connaissances. Je
remercie également Nick, Penny, Louis, Alexandre, Éveline, Bevan et Stéphane pour leur aide. Je
souhaite particulièrement remercier Chantal pour nous avoir toujours épaulé lorsque nous avions
le moindre problème ainsi que Émilie qui, bien que ma stagiaire par alternance, m’a énormément
appris sur la pédagogie.
En ce qui concerne la vie universitaire, la liste de mes remerciements serait trop longue
pour être nominative et je le regrette, mais je tiens à remercier très particulièrement les étudiants
de l’IAF ainsi que les membres de l’association étudiante qui ont cru en moi pour les représenter
pendant ces deux dernières années. Je remercie également les directeurs de l’institut, Mrs Charles
Dozois et Alain Fournier ainsi qu’Anne Phillipon pour leur écoute face aux nombreux problèmes
étudiants qui se sont présentés et pour la confiance qu’ils ont eue en moi. Je remercie aussi mes
compagnons du congrès Armand-Frappier pour la fantastique aventure que cela a été.
J’aimerais finalement remercier les membres de ma famille qui m’ont encouragé et
soutenu durant mes études universitaires. Pour finir, je remercie infiniment Soizic, ma compagne,
pour son aide, son soutien et sa patience. Elle a plus que quiconque cru en moi et a toujours su
trouver les mots pour m’encourager à persévérer. Elle m’a été une source d’inspiration
quotidienne. Cette thèse ne serait pas sans elle.