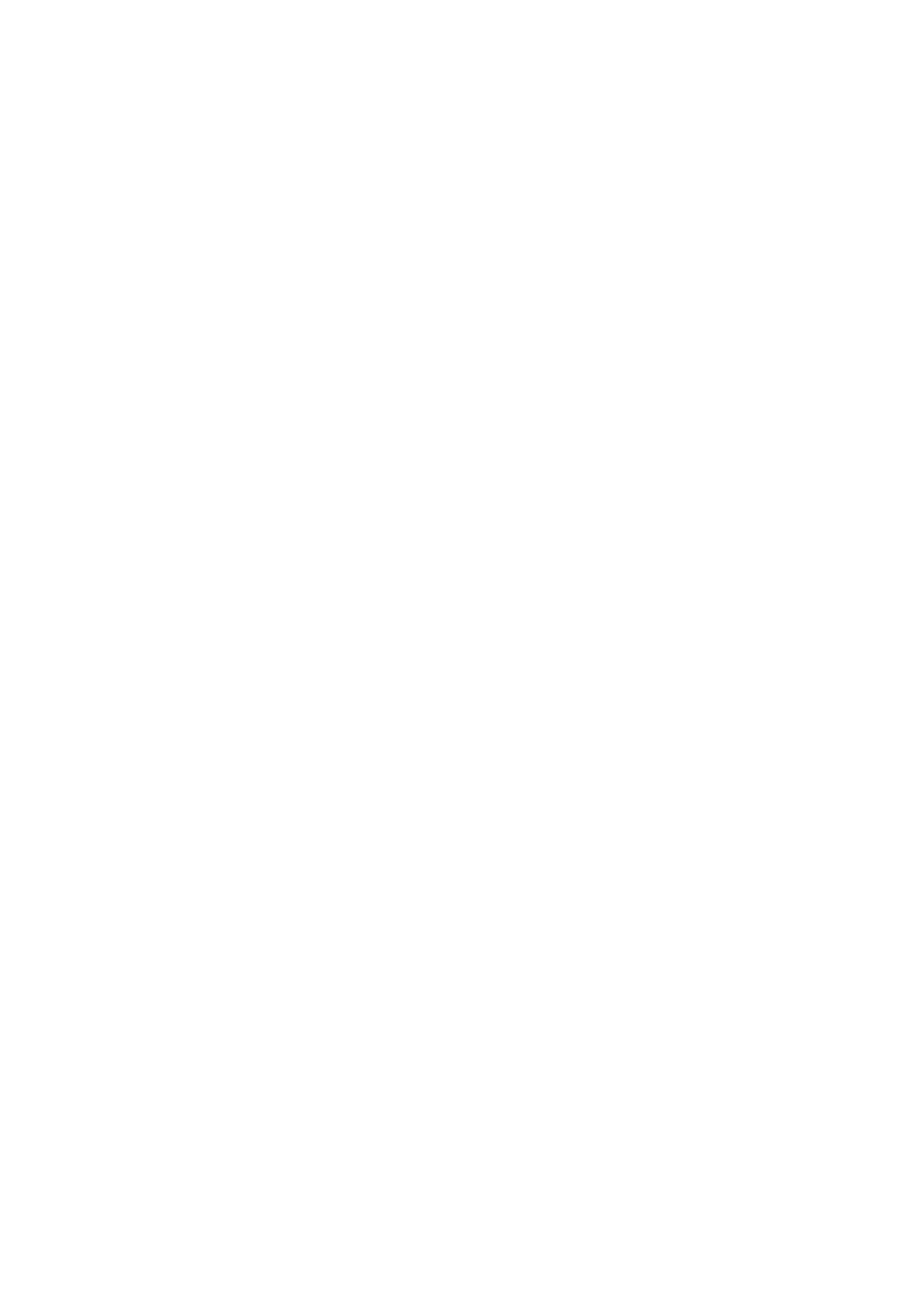
4
Les travaux exposés dans ce mémoire ont été réalisés entre septembre 2004 et octobre
2007 au Laboratoire de Chimie, Catalyse, Polymérisation et Procédés dans l’équipe de
Chimie OrganoMétallique de Surface, unité mixte CNRS-CPE Lyon. Je suis reconnaissant
envers Monsieur Gérard Pignault, directeur de CPE Lyon, pour m’avoir accueilli dans ses
locaux.
Mes remerciements vont tout d’abord à Monsieur Jean-Marie Basset, Directeur de
Recherche au CNRS, qui a bien voulu m’accueillir au sein de son laboratoire.
J’adresse mes plus vifs remerciements à Monsieur Yves Chauvin, Directeur de
Recherche honoraire à l’Institut Français du Pétrole, agissant en qualité de co-directeur. En
dépit de son éloignement, il a fait preuve d’une grande disponibilité et a été une source
constante de conseils et de réflexions. Je lui suis très reconnaissant de sa contribution au
développement de ce projet et de m’avoir fait profiter de ses connaissances et de sa grande
expérience en chimie.
Je tiens à remercier Madame Catherine Santini, Directeur de Recherche au CNRS, qui
a encadré mes travaux de thèse. Je la remercie tout particulièrement pour l’aide et le soutien
qu’elle m’a apportés durant ces trois années.
Que Madame Olivier-Bourbigou, chercheur à l’Institut Français du pétrole et
Messieurs Marc Lemaire, Professeur à l’Université Lyon 1, Bruno Chaudret, Directeur de
Recherche au CNRS, Jean-Pierre Simonato, chercheur au Commissariat à l’Energie
Atomique et David Cole-Hamilton, Professeur à l’Université de St Andrews, soient vivement
remerciés de l’honneur qu’ils m’ont fait en acceptant de juger ce mémoire.
Ce travail a été effectué en collaboration avec le Commissariat à l’Energie Atomique,
l’Institut Français du pétrole et la société Rhodia. Je tiens à remercier ces partenaires pour
l’aide qu’ils ont portée à la progression de cette recherche.
Je tiens également à remercier Monsieur Bernard Fenet, le professeur Denis Bouchu,
le professeur Agilio Padua pour leur aide .
Que tous les membres du laboratoire trouvent dans ces quelques lignes l’expression de
ma plus profonde gratitude. Je pense en particulier aux étudiants en thèse ou en DEA avec
lesquels j’ai partagé tout ou une partie de ces trois années.